The importance of technology cannot be underestimated in the context of industrial organizations and its implications on academia and society as a whole. Technology plays a key role in the energy industry as it revolves around long-term drivers such as climate change and the balance of supply/demand curves.
The future of technology is conventionally predicted using foresight tools such as Delphi; political, economic, sociological, technological, legal, and environmental (PESTLE) analysis; cross impact analysis; expert panels; horizon scanning; modeling; mapping; and extrapolation. The article is written from the perspective of horizon scanning in the hydrogen sector. This resulted in the identification of three progressing areas (Fig. 1).
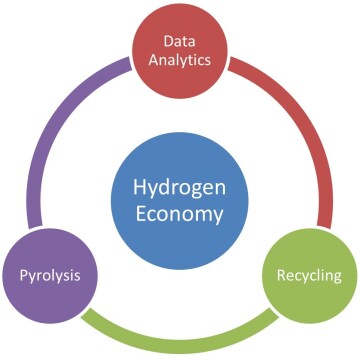
This article is intended to help energy engineers and researchers advance careers in these areas with capstone projects, field familiarization, internships, research initiatives, and trading.
Data Analytics
Big data and its analytics are pathways to success in research and business. Green hydrogen as an emerging sector can benefit from analytics by its integration into the core hydrogen market sector. There are three ongoing developments in this section.
Evaluation of Green Hydrogen Reserves and Renewable Energy Resources. Because renewable electricity is needed for green hydrogen production, an analytical model can generate a classification system by mapping confidence and uncertainties locationwise for hydrogen production and renewable energy. This mapping can be used to encourage investments and developments in both of these sectors (Green Hydrogen Solutions).
Application for Green Hydrogen Value Chain. A mobile-application-based algorithm can assist in the pricing structure, process efficiency, and market assessment of using green hydrogen facilities in buildings for heating and power, transportation, industrial chemicals, shipping, aviation, and steel in developing countries. This app can help users entering the hydrogen economy get acquainted with existing market/technology/pricing scenarios across the value chain (Green Hydrogen Solutions).
Predictive Analytics for Electrolysis. The electrolysis process has various elements such as electrode kinetics, electrochemical reactions, current and voltages for cell efficiency, the chemistry of electrolytes, additives, and catalysts. A smart control system is designed such that it optimizes and maintains machine efficiency with real-time data analysis. The model uses the same data for drawing predictions to reduce unintended shutdowns and equipment failures. This can further reduce unwanted costs, enhance plant efficiency, and maximize hydrogen production (Envision Digital; Zeng). The following are key developers of analytics in green hydrogen.
- Nestity, US
- Empati, UK
- Envission Digital, Singapore
- Green Hydrogen Solution
Pyrolysis
The mature technologies for hydrogen production from methane are steam methane reforming and autothermal reforming. Both of these processes are industrially recognized with high technology readiness levels releasing almost 11 kg of CO2 for 1 kg of hydrogen production (Oni et al. 2022).
Blue hydrogen pairs mature and competitive processes (methane reforming) with a nascent energy-intensive technology [carbon capture and storage (CCS)]. Operating at maximum carbon-capture rates, blue hydrogen technologies still emit 4–6 kg of CO2 for 1 kg of hydrogen production. Moreover, CCS is highly linked with carbon price/tax. The carbon-capture rate benchmark will depend on the costs of capturing CO2 weighted against the carbon tax. Considering the limitations of blue hydrogen and understanding the role of natural gas in the transition from a gray to a green hydrogen economy led to the neutral philosophy of turquoise hydrogen (Oni et al. 2022).
Turquoise hydrogen comes from natural-gas decomposition. This method involves the thermal pyrolysis of methane into solid carbon and gaseous hydrogen molecules. This is one simple endothermic process with the near elimination of greenhouse-gas emissions. The technology’s readiness level is at the laboratory scale because of its very strong carbon/hydrogen bonding (440 kJ/mol). This reaction is only possible in the absence of a catalyst at temperatures higher than 1200°C (Ashik et al. 2015).
Turquoise Hydrogen Major Progress Areas
Catalysts. Methane cracking to produce hydrogen and carbon requires temperatures above 1200°C. This temperature can be lowered by carrying out the decomposition reaction in the presence of a catalyst. Significant efforts are being made to search for a suitable catalyst. The catalysts should be resistant to choking and have good stability. Several metals (nickel, iron, cobalt), carbon, and nanocatalysts are in the development and testing stage (Banu and Bicer 2021).
Heating Source. Methane decomposition is a moderately endothermic reaction. There are many reports on the use of an electric furnace as a heating source, but, for environmentally friendly options, the focus has shifted to the use of concentrated solar energy, plasma, renewable power, or a molten-metal bath as heating sources (Abbas and Daud 2010).
The following are some of the key developers in turquoise hydrogen:
Research Institutes
- King Abdullah University of Science and Technology, Saudi Arabia
- University of Science and Technology, Nanjing, China
- University of Sheffield, UK
Businesses
- HIIROC, UK
- Ekona Power, Canada
Recycling
Three different kinds of electrolyzers are available for the production of green hydrogen. Proton exchange membrane (PEM) electrolysis is the only one that uses platinum-group metals (PGMs) as electrocatalysts. It uses platinum for the hydrogen-evolution reaction at the cathode and uses iridium oxide for the oxygen-evolution reaction at the anode (Kumar and Himabindu 2019).
PEM electrolysis has several advantages such as high-pressure hydrogen, compact design, efficient production in terms of purity, effective membrane separation and conductivity, small footprint, compatibility with renewable power, and a low operating temperature. This makes it appealing to the world market (Kumar and Himabindu 2019), resulting in the majority of new investment and research in the direction of PEM electrolysis. Top downloaded research works on Science Direct in the International Journal of Hydrogen Energy are on PEM electrolysis.
The world has significant PGM deposits in various geological structures in South Africa, Russia, the US, and Canada. PGM mining is becoming less appealing because of the high risks of advanced exploration, mining techniques, price vulnerability, high capital expenditure, and substantial negative environmental impacts (Koek et al. 2010). The gross demand continues to rise, however, in the autocatalyst, chemical, dental, electronics, and jewelry sectors. This increase in demand is without the advent of green hydrogen production from PEM electrolyzers, which is projected to see significant growth in the future. Countries with few of no reserves are vulnerable to supply and price risks. Recycling PGM uses almost 98% less energy than mining and uses considerably less water. Recycling PGMs could be almost 10–13 times less expensive than mining from ores (Hope 2018, Visser 2014). Thus, recycling is the only way to secure a green future and achieve green hydrogen production targets.
Technological Solutions for Recycling PGMs
The following solutions are from Chagnes et al. 2016, Diac et al. 2020, Ding et al. 2019, Karim and Ting 2021; Quijada-Maldonado et al. 2020, and Moschovi et al. 2020.
Pyro Metallurgy
Process Description: This energy-intensive thermal treatment of the equipment/membrane uses a heated reactor for physical and chemical conversion. The process starts with smelting, fluxing, and a reduction reaction at high temperatures.
Future Advancements: Pyrochemical methods include thermal and chemical treatment. One such example is chlorination at high temperatures. These technologies have a high recovery rate but are currently at the laboratory scale.
Hydro Metallurgy
Process Description: This is a chemical-based method for leaching out the metal and then, through precipitation, ion exchange, or solvent extraction, concentrating PGMs from solution. It is widely used for PGM recovery.
Future Advancements: Ionic liquids are greener options than the current organic solvents used in leaching. It has high extraction capabilities, higher selectivity, and allows for easy recycling of the liquids for reuse, with further investigation looking at maintaining its viscosity and dilution issues.
Electrochemical Dissolution
Process Description: Dissolution of platinum from electrolytes takes place in presence of chloro complexes. Chlorides use a degradation mechanism to separate platinum group elements from PEM electrolyzers.
Future Advancements: The process of dissolution requires suitable pH, temperature, and voltage. Further research is required to achieve high recoveries and reactivity at moderate temperature without the liberation of toxic gases.
Biometallurgy
Process Description: This method involves pretreatment by microbe oxidation reaction, followed by leaching and adsorption of metal extracts on living or dead biomass and, lastly, mineralization of the recovered material.
Future Advancements: This young technology requires microbe growth for leaching and is increment in kinetic and reaction time.
Recycling initiatives are being taken by the following:
Research Institutes
- Institute for Nonferrous Metallurgy and Purest Materials, Germany
- Danish Technological Institute, Denmark
Businesses
- Hytech Recycling, Spain
- Recycalyse, Denmark
- Hydrogen Centre, Austria