For many outcomes, roughly 80% of the consequences come from 20% of the causes, as stated by the Pareto principle. The Groningen field in the Netherlands, the largest gas field in Europe, has grappled with production-induced seismicity since 1991, prompting the Dutch government to cap annual gas production in 2014 and announce a halt to gas extraction in October 2023, and then announced it will allow minimal production under certain circumstances in January 2024.
This article evaluates the geomechanical impacts of depleting and repressurizing such gas reservoirs, while proposing laboratory measurements to enhance understanding of geomechanics.
Withdrawal or injection of fluid in large volume from the subsurface during activities such as oil and gas extraction, geothermal energy exploitation, or CO2 storage, changes the reservoir pore pressure which subsequently perturbs the insitu stress state and causes alteration. This results in potential surface-elevation changes. Surface-elevation changes and stresses can manifest as fault slippage, subsidence, and reservoir compaction.
With such potential environmental risks, it is important to identify how the subsurface responds during these activities to know how best to mitigate or manage the situation at hand.
Netherlands Energy Industry
Prior to the discovery of large amounts of gas by the Slochteren-1 and Delfzijl-1 wells in 1959 and 1960, respectively, the Dutch government relied on coal mines and oil for provision of energy. The absence of gas infrastructure and market generally made gas exploration an unattractive business. However, the discovery of large gas reserves changed the story. This discovery not only led to the closure of coal mines, but it also facilitated the development of smaller gas fields, both onshore and offshore, that were previously deemed noncommercial. It was considered to produce gas from the smaller fields and defer production from the Groningen—delineating it as a strategic reserve to meet peak demand only—but the forecasted pressure behavior of the field showed great decline which made this proposal unrealistic.
Groningen Gas Field
Groningen gas field, operated by Nederlandse Aardolie Maatschappij B.V. (NAM), started production in 1963 following its discovery in 1959 by the Slochteren-1 well. The Slochteren Sandstone, part of the Rotliegend reservoir which feeds the field, exists at a depth of 3 km. The caprock for this gas-bearing formation is the Ten Boer Claystone unit and the Zechstein evaporite series.
Depletion
In the Netherlands, it was required by law to monitor the level of subsidence in gas fields prior to commencement of production and then all the way to the end of life of the field (Thienen-Visser and Breunese, 2015). For the Groningen field, this was essential, most importantly due to its proximity to sea level. Additionally, it was crucial considering the potential effect of subsidence on groundwater, which greatly influenced agricultural activities in the area. In view of this, optical leveling survey was the first technique deployed on the field in 1964 to keep track of subsidence.
Interferometric synthetic aperture radar satellite systems and global positioning systems were later deployed in 1996 and 2013, respectively. The former was for consistency check of data from optical leveling survey, and the latter was for real-time monitoring of subsidence following the largest seismic event in the field. Until 2001, a maximum of five seismic events occurred yearly with magnitudes of about (ML ≥ 1.5). However, this number increased from 2003. On 16 August 2012, the Huizinge earthquake, the largest-magnitude seismic event of ML 3.6 and destroyed properties, was recorded. This occurrence necessitated a government study to understand the reasons for these events.
Following increasing seismic events with gas production from the field highlighted as the possible cause, especially the center of the field where reservoir compaction was the greatest, the Dutch government in 2014 mandated a production cut.
What Happens in the Reservoir During Gas Production?
In a hydrocarbon reservoir, it is expected that the least horizontal stress declines as the reservoir pressure declines (Segall and Fitzgerald, 1998). During gas production, the pressure of gas in the pores of the reservoir decreases; this reduction causes reservoir rocks to undergo slight contraction which stresses the surrounding rocks. Vertical effective stress in turn increases during production due to constant lithostatic pressure resulting in contraction above the reservoir in the horizontal direction, inducing fracturing, fault slip, and compaction which is seen as subsidence at the surface. Illustration of stress response to pore pressure change is shown in Fig. 1.
With the Groningen field being in an extensional environment, production will favor normal faulting and dilatant fracturing in the reservoir vicinity (Fig. 2).
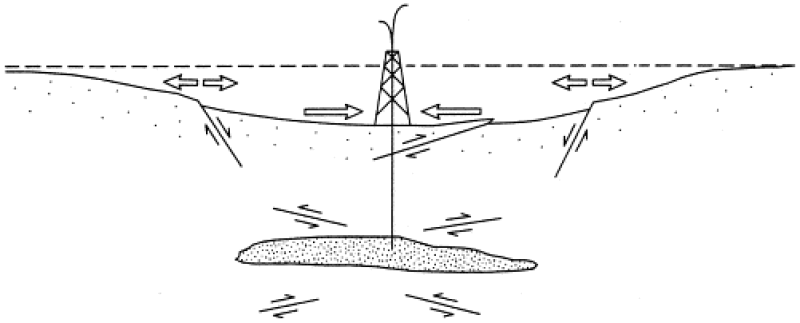
Likely Deformation Mechanisms
The deformation mechanism in Groningen field is likely a combination of porosity reduction, grain rearrangement, and grain crushing. As the vertical effective stress increases during production, the contact area between mineral grains that make up the rock increases, compressional forces cause grains to break, and all these cumulatively cause grain rearrangement, closure of pores, and grain-size reduction (Spiers et al., 2017).
Laboratory Measurements
The following laboratory measurements can help increase the understanding of geomechanics in Groningen.
- Triaxial compression test: To determine the rock strength, deformation behavior, and controlling mechanisms of the producing reservoir from cores. The consequence of grain-size distribution and the porosity difference can also be investigated.
- Strain gauge: By directly measuring elastic strain, the importance of energy stored elastically and that dissipated during compaction is identified.
- Acoustic emission: To identify microcracks and other small-scale fractures within the rock.
- X-ray diffraction analysis: To identify microscale physical processes and provide information on the mineralogy and crystal structure of the rock.
- 4D X-ray microtomography: This can help quantify how strain propagates and accumulates as deformation occurs by allowing imaging of samples during active deformation under in-situ pressure and temperature conditions.
Repressurization
Produced gas from the Groningen field contains about 14% nitrogen. Because of this, it is not surprising to see it being considered for nitrogen storage. Initially, the reservoir pressure of the field, which was same across the field at reference depth of 2875 m, was about 35 MPa. This dropped to 8 MPa over the span of 55 years of gas production. The recorded reservoir temperature was 373.15°K. Although gas expansion drive is the primary production mechanism, further pressure support from aquifers and compaction-induced volume reduction also exist (Jager and Visser, 2017).
Injection of CO2
Injection of fluids into the subsurface changes the pore pressure of the formation resulting in alteration of the effective stress state of the formation. This effective stress state controls mechanical rock deformation and rock failure. If the CO2 being injected is supercritical or liquid, there are additional chemical, hydrological, and mechanical processes that can affect the mechanical properties of the storage site. CO2 injection also locally perturbs temperature. As CO2 dissolves in brine, acidic fluid results and this affects the rock-fluid chemical equilibria and stress of the field where it is injected. Porosity and permeability could also change due to the reactivity of CO2-saturated brine.
Potential geomechanical consequences that can emanate from repressurizing the Groningen field:
- In the Slochteren sandstone reservoir and Zechstein salt caprock, pre-existing faults could be reactivated by the injection of CO2 which can change pressure of fluid in the pores of fault planes.
- With the field already undergoing reservoir compaction, there is a potential to cause CO2-enhanced creep processes which further increase compaction rate. Reservoir heave can also emanate from the reservoir’s poroelastic response.
- Zechstein salt caprock integrity may be a concern if very cold CO2 is injected at a very high rate which could cause hydraulic fracturing near the point of injection.
Induced Seismicity
Due to depletion, stresses change inside and outside the reservoir. How much they change depends on the degree of pore-pressure changes and rock properties. Slippage along weak layers, fault reactivation, or tensile/shear failure can result from stress change. Therefore, knowledge of the stress orientation in relation to orientation of pre-existing fault is needed for analyzing the slip tendency of faults.
A methodology to evaluate if a stress state will lead to formation shear failure involves the use of the Mohr-Coulomb criterion, which is defined by cohesion and the friction angle. Above the failure line, the material is mechanically unstable, and reactivation of fault leading to induced seismicity is more likely to take place. Below the failure line, the material is stable. Also, if the Mohr’s circle remains below the failure line, the material is in shear failure; otherwise, it is in an elastic state.
An illustration showing the use of Mohr’s circle is seen in Fig. 3.
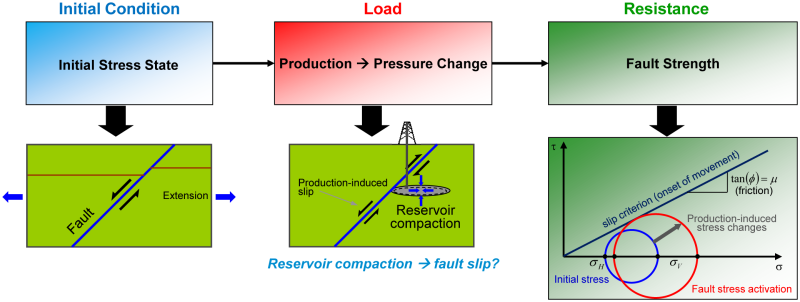
Conclusions
Unlike many other gas fields that may have deeper reservoirs, the Groningen field is relatively shallow, with the gas reservoir located close to the surface. This makes the impact of gas extraction on the overlying rock formations more pronounced and contributes to faster and more extensive subsidence. Characterized by many normal faults, it is important that CO2 injection should not be in the vicinity of faults to avoid direct pore-pressure increase in faults. If CO2 enters the fault plane, the tendency of reactivation increases as the fault plane is lubricated by the increasing pore pressure.
For Further Reading
The Exponential Rise of Induced Seismicity with Increasing Stress Levels in the Groningen Gas Field and its Implications for Controlling Seismic Risk by S. Bourne, Shell; S.J. Oates, J. Van Elk, Shell.
Geology of the Groningen Field – An Overview by J. De Jager, C. Visser, NAM.
Reassessment of the Probability of Higher-Magnitude Earthquakes in the Groningen Gas Field by A. Muntendam-Bos, Delft University of Technology; J.A. de Waal, State Supervision of Mines.
SPE 174942 Geomechanical Analysis to Evaluate Production-Induced Fault Reactivation at Groningen Gas Field by P. Sanz, ExxonMobil; S. Lele, K. Seares, EMURC, et al.
A Note on Induced Stress Changes in Hydrocarbon and Geothermal Reservoirs by P. Segall and S. Fitzgerald; Stanford University.
Peterhead CCS Project, Shell UK Ltd.
New Approaches in Experimental Research on Rock and Fault Behavior in the Groningen Gas Field by C. Spiers, S. Hangx, and A. Niemeijer, Utrecht University.
Induced Seismicity of the Groningen Gas Field: History and Recent Developments by K. Thienen-Visser and J.N. Breunese.