This paper provides a review of recent technology advancements and addresses practical considerations associated with drillpipe and drillstem components for extreme drilling applications. Recent developments and enhancements in these connection designs, including new higher-strength materials, advanced thread forms, and associated improvements in torsional strength and fatigue performance, are presented. The paper discusses the engineering solutions implemented to overcome the high forces, slip-crushing concerns, and material-strength and toughness considerations for these critical applications.
Third-Generation Double-Shoulder Connection
Since the introduction of the second-generation double-shoulder connection (DSC), the industry trend has continued toward deeper and longer-reach wells, which has dictated the need for drillpipe connections with enhanced mechanical and dimensional characteristics coupled with improved makeup/breakout speeds.
Consequently, a project was commissioned to design, analyze, laboratory test, and field trial the industry’s first third-generation DSC. A key objective of the project was to improve connection makeup/breakout speeds significantly. Mechanical and hydraulic gains were also dictated on the basis of the industry’s trend toward deeper and longer-reach wells.
Design Philosophy. One of the primary philosophies underlying the development of third-generation DSCs was the concept that “one design does not fit all.” This philosophy suggests that a thread form optimized for 6⅝-in. drillpipe may not be optimal for 2⅜-in. drillpipe. In fact, optimized thread forms for each of these sizes differ substantially.
5- to 5⅞-in. drillpipe sizes represent common sizes for offshore, deepwater, and higher-profile programs such as extended-reach drilling. Connection designs focused on speed of makeup and more-streamlined connections for increased hydraulic performance.
For the large 6⅝-in. drillpipe size commonly run in elevated-spread-rate projects such as deep and ultradeep water, speed of makeup is a primary design objective. In addition, makeup torques can be excessive, at times surpassing the capacity of the rig equipment. Design parameters must be balanced to reduce the makeup torque and improve hydraulic performance.
Connection Design. Third-generation DSCs differ from first- and second-generation DSCs in several ways. One of the primary differences is the addition of a dual-start, twin-lead, or double-start thread (Fig. 1 above). The design components listed in this subsection are discussed in greater detail in the complete paper. In addition to double-start threads, these include the following:
- Dual-Radius Thread Form. Thread-root radius is lengthened and radically improved with the dual-radius thread form in third-generation DSCs.
- Optimized Taper. Taper defines the cross-sectional area at the secondary shoulder providing the improved torsional strength and controls the stabbing depth of the pin to the box, affecting makeup and tripping speeds.
- Material Strength. American Petroleum Institute (API) tool joints are produced with specified minimum yield strength (SMYS) of 120,000 psi. During development of third-generation DSCs, a program was commissioned to develop 130,000-psi-SMYS tool joints to meet the stringent toughness requirements of many proprietary manufacturing specifications.
Primary benefits of third-generation DSCs include time and cost savings, increased torque capacity, larger equivalent hydraulic inner diameters, improved clearance and fishing ability, reduced failure risk, and extended life.
Landing-String Development
Deepwater- and ultradeepwater-well designs continue to drive the requirement for higher-tension-capacity landing strings. Water depth and total depth are increasing, and stepouts are being extended. This, combined with the often narrow margin between pore pressure, mud weight, and fracture gradient, is causing well designers to set more intermediate casing strings and in turn is pushing large-diameter, heavy casing strings to deeper setting depths in order to maintain hole size and reach the intended hydrocarbon targets.
Initially, casing, liners, and offshore casing strings set in subsea wellheads were simply run on the drillpipe that was used to drill the well. As setting loads increased, however, initial fit-for-purpose solutions were developed with increased load capacities targeted toward anticipated running loads for specific areas or projects. During this period, landing strings built from casing were also used. It quickly became apparent that drillpipe landing strings offered significant advantages:
- Rotary-shoulder connections are rugged and robust.
- Conventional drillpipe-handling equipment can be used.
- A drillpipe landing string can incorporate connections with tensile capacity that exceeds that of the pipe body.
As ultrahigh-capacity landing strings were developed, slip crushing was quickly identified as a major design and manufacturing obstacle. With the slips currently available, the slip-crushing resistance for the pipe body is less than its axial tensile capacity. To address this issue, a special thick-walled section was provided in the slip-gripping area. Dual-diameter tool joints were used to increase elevator capacity.
To achieve a lifting capacity of 2.5 million lbm, a state-of-the-art landing-string assembly is required (Fig. 2).
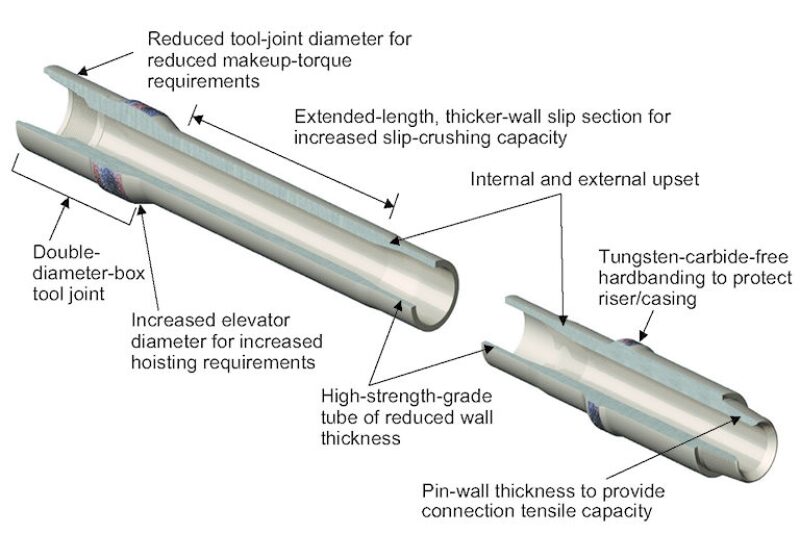
Pipe Body
The tensile capacity of the pipe body is defined as the pipe-body yield at the SMYS, or grade, times the pipe-body cross-sectional area. There is benefit from matching the landing-string-pipe diameter to the drillpipe diameter used on the rig, where possible.
Early landing-string-pipe bodies were commonly produced from material of S-135 grade. Now, there are proven high-strength proprietary grades available with SMYS of 140, 150, and 165 ksi. Use of these grades provides increased lifting capacity of up to 22%.
For 6⅝-in.-diameter V-150 pipe, 1.125-in. wall thickness is required for the pipe-body tensile rating at 90% remaining-body-wall to meet the 2.5‑million‑lbm rating. By using a 165,000-psi-SMYS pipe, the wall thickness can be reduced to 1.000 in., resulting in a 5% decrease in string weight.
Development of UD-165. A drillpipe grade with minimum yield strength of 165 ksi was developed to meet the needs of both high-capacity landing strings and high-capacity drillstrings required for drilling ultradeep wells, and the needs of high-strength-to-weight drillstrings required to reduce tensile and drag load in ultraextended-reach wells.
Heavy-Walled Slip Section (HWSS). Slip-crushing capacity can be the primary design factor for landing strings because it is less than the tube tensile capacity. In the deepwater Gulf of Mexico, some slip-crushing failures have resulted in catastrophic events involving the loss of casing strings to the seafloor. One way to increase slip-crushing capacity is through the pipe design. The HWSS provides a thicker wall in the slip-contact area (Fig. 3).

Weld Strength. For the 2.5-million-lbm landing string, the expected weld yield strength would be 125,000 psi or higher. The required weld yield strength calculates to 122,657 psi, which is below the 125,000-psi minimum and is therefore acceptable.
Design of a safe and functional 2.5-million-lbm landing string was accomplished, although it pushed manufacturing capabilities to their limits.
First Fully Sulfide-Stress-Cracking-Resistant System
As the severity of sour-drilling applications has increased, the need for drillstem materials resistant to sulfide stress cracking (SSC) has become acute. Sour-service drillpipe has been available for some time, and though the metallurgy is not specifically controlled by National Association of Corrosion Engineers International standards, these tubulars and tool joints are often evaluated in accordance with those standards. The friction welds joining the upset tubulars and tool joints were not resistant to SSC and were not evaluated. This has been acceptable for many sour-drilling applications because the weld is not the mostly highly stressed region of the drillpipe joint and because the operator has a certain degree of control over the environment through the drilling-fluid properties and additives. As more-severe environments with higher hydrogen sulfide (H2S) concentrations were identified for exploration and development, it became apparent that a fully SSC-resistant drillpipe system, including the friction welds, was necessary.
Sour-Service Drillpipe
SSC caused by the presence of H2S gas in downhole drilling environments has led to the development of sour-service drillpipe, which is engineered to have resistance to SSC. The weld area of sour-service drillpipe has not been SSC tested in the past, and there have been no documented SSC failures in the weld zone of sour-service drillpipe. There are several factors that make an SSC failure in the weld zone of sour-service drillpipe unlikely. The region on both sides of the weld has a cross section much larger (1.5 to 2.0 times) than that of the tube. This larger weld-area cross-section means that the stress experienced in that area is less by the same proportion. Also, it is generally possible during drilling operations to control the well environment and help prevent SSC failure of the drillpipe and weld zone.
On the other hand, the operating environment for some critical sour applications cannot always be controlled, and direct and prolonged exposure to H2S can occur. Consequently, it became apparent that an SSC-resistant friction weld was required for these critical sour applications.
Friction-Type Welds and SSC Resistance
During friction welding, heat is generated by mechanical friction between a rotating tool joint and a stationary upset tube. At forging temperatures, a lateral force is applied to displace and fuse the components plastically. The weld area is effectively forged, resulting in a high-strength weld. The weld area is then austenitized, quenched, and tempered to produce a final tempered martensite microstructure. Careful weld-process control and heat treatment are required to produce weld-area SSC resistance in friction-type welds.
For a discussion of SSC-testing parameters, as well as tensile-test and bend-testing results, please see the complete paper.
Downhole-Heating Failures
Downhole-friction-generated-heating failures are another problem experienced in a number of deepwater drilling applications. Directional drilling followed by long, potentially high-angle, deviated sections can create conditions conducive to downhole heating.
The consequences of downhole heating can be severe, often resulting in axial separation of the drillstring, creating potential well-control safety issues and need for costly fishing jobs and other remedial efforts.
Three conditions are required for the production of friction heating: side loading, rotation, and a sufficient coefficient of friction between the surfaces. These conditions are met in several ways, including, but not limited to, rotating in an excessively severe dog-leg, continued rotation while in a stuck situation, drilling in an interval that has a high number of wellbore-trajectory corrections, and drilling when there is formation sloughing or insufficient mud flow that fails to remove cuttings (packing off).
Identification Features. Field observation assisted by magnetic-particle inspection, if available, can identify downhole heating as a likely failure cause. Metallographic- and microscopic-image analyses are not possible in the field; however, they are necessary to determine conclusively that failure was the result of downhole heating. Below is a checklist, for use in the field during a failure analysis, of the main features of downhole-heating failure:
- Smooth, shiny surfaces from friction wear.
- Blackened and charred inner surfaces near the location of the failure or thick blackened sludge formed by burning of drilling fluids.
- Exaggerated necking and elongated necking of the region near the failure.
- Flat fracture faces.
Downhole-overheating failures typically occur in or near the transition area between the tool joint and drillpipe upset or heavyweight-drillpipe tube (18 or 35° shoulder area) because this area can be caught by a ledge, key seat, or other wellbore obstruction during drilling operations.
A case history involving an extreme example of downhole-friction-generated heating in 5-in. 19.50-lbm/ft Z-140 drillpipe is covered in the complete paper.
This article, written by JPT Technology Editor Chris Carpenter, contains highlights of paper SPE 170566, “Advanced Technologies and Practical Solutions for Challenging Drilling Applications,” by M.J. Jellison, SPE, NOV Grant Prideco, and A. Chan, Workstrings International, prepared for the 2014 SPE Asia Pacific Drilling Technology Conference and Exhibition, Bangkok, Thailand, 25–27 August. The paper has not been peer reviewed.