To estimate reserves, optimize well recovery, and place future development wells more accurately, operators require increasingly detailed understanding of complex oil and gas reservoirs. High-resolution geological data from the borehole—core samples and wireline microresistivity images—are essential to fully characterize reservoir architecture, guide and constrain reservoir models, and make timely decisions with precision and confidence.
In unconventional resource plays, for example, geologists need to observe complex natural fracture systems, measure fracture density and direction, determine in-situ stresses, and calculate pressures required to initiate and propagate optimal hydraulic fracturing. In high-cost deepwater environments, exploration teams need to interpret a wide variety of sedimentary facies. Reservoirs often consist of thinly laminated sands or channels, which demand high-definition methods for visualization and interpretation. Typically, deepwater formations exhibit very low resistivities—from 1 ohm-m in shales to as low as 0.2 ohm-m in water-bearing sands.
Operators often use whole cores to identify subtle sedimentary facies, but cores can be expensive and time-consuming to acquire. Thus, core samples are usually obtained only in select wells and in the most critical intervals. The resulting lack of geological detail can adversely affect reservoir analysis, interpretation, and modeling.
On the other hand, microelectrical borehole images can be acquired continuously over the openhole interval at any depth or in any formation, with relative ease. Until recently, high-definition borehole imaging has been possible only in electrically conductive water-based muds (WBM). However, most deepwater wells and many unconventional shale wells today are drilled with high-performance, nonconductive oil-based muds (OBM).
Legacy OBM borehole imaging tools, which were introduced in the last decade, are a significant technical advancement over the preceding dipmeter tools but still exhibit certain limitations. For example, spatial image resolution in OBM is nowhere near the quality obtained from WBM imagers. The lack of circumferential coverage of the borehole leaves large gaps that must be filled by inference.
OBM imagers also rely on complex physics to measure formation resistivity beyond the nonconductive mud barrier. These may create geologically nonrepresentative artifacts such as shadow beds that hinder the visualization of formation geology. Largely because of electrode geometry, conventional OBM borehole imaging tools may have low sensitivity to features that run nearly parallel to the wellbore.
Finally, the mechanical design of all existing borehole imaging tools allows them to record data only while exiting the hole. This limitation increases the occurrence and severity of stick-and-slip, which adversely affects final image quality.
For these reasons, geoscientists are often subject to uncertainties about reservoir texture, sedimentology, depositional environment, and important structural elements such as the recognition and orientation of faults and fractures. The industry has long recognized the need for an OBM-adapted imaging technology that can provide resolution and borehole coverage comparable to the best imagers in WBM environments.
Imaging in Nonconductive Mud
To offer OBM microresisitivity imaging with resolution as high as—and circumferential coverage greater than—leading WBM imagers, Schlumberger recently developed the Quanta Geo photorealistic reservoir geology technology. In more than 60 field-test jobs, the new tool generated high-definition images in OBM formulations with oil/water ratios as low as 60/40 and as high as 90/10, and in environments ranging from high-resistivity carbonates to shales and low-resistivity clastics.
The tool represents a complete re-engineering of earlier imaging technology, delivering nearly total borehole coverage—98% in 8-in. holes—and creating core-like, photorealistic representations of reservoir geology. With a new sonde design, the system is the industry’s first borehole imaging technology capable of downlogging, or recording high-resolution image data, while being run into the hole.
In addition to fully digital electronics operating at much higher frequencies, the new OBM imager has a unique mechanical design and operates with completely new measurement physics and more microelectrodes than other borehole imaging tools.
The tool has eight fully independent double arms equipped with eight imaging pads to maintain the sensor array in close contact with the formation under any borehole conditions. Each pad can swivel 15° around its long axis and can change pitch angle because the top and bottom arms operate autonomously. Thus, each pad can maintain reliable contact with the borehole wall, even in highly deviated wells and poor borehole conditions.
When the tool is slightly eccentered, advanced processing software maps image data to the correct position within the borehole. Deploying pad arms independently using spring force generates less friction than the legacy technology. Thus, operators can obtain high-resolution images during the tool’s descent, while minimizing the stick-and-slip effect on image quality.
Unlike standard WBM borehole imaging, all measurements of the OBM tool take place on the pads. Components include an injector in the center of each pad and two large return electrodes (Fig. 1 above). The injector consists of an array of 24 microelectrodes, surrounded by a guard ring. With eight pads, the new OBM imaging tool has 192 microelectrode buttons—three times more than the legacy OBM imagers. Each button has a surface area of only 10.8 mm2, which is smaller than the button surface area of the best WBM imagers.
High-frequency AC voltage is applied between the injector and the returns, causing current to flow out from each button, through the OBM into the formation, and back into the return electrodes. The tool injects a blend of two high frequencies in the megahertz range—three orders of magnitude higher than previous OBM imagers, which reduces the effect of nonconductive mud on the measurement. Advanced phase-sensitive signal processing separates the remaining mud impedance from that of the formation, resulting in high-quality photorealistic images that visually represent formation geology.
The new simplified electrode geometry eliminates artifacts associated with the previous generation of OBM imagers. For example, the tool is equally sensitive to vertical and horizontal features. Thus, it can measure fractures at any inclination to the wellbore, while reducing sensitivity to artifacts such as desiccation cracks, which obscure formation geology. At a maximum logging speed of 3,600 ft/hour, the new OBM imager can achieve a sampling rate of 0.2-in. At 1,800 ft/hour, it can achieve a 0.1-in sampling rate. Finally, it can measure a wide range of formation resistivities from 0.2 ohm-m to 20,000 ohm-m—and thus is far more versatile than other OBM imaging technologies.
Case Studies
Targeting a deepwater slope system in the Gulf of Mexico, an operator was drilling with high-performance OBM using a 9⅞-in. bit. To understand the commercial potential of the prospect, the geologists needed to interpret tectonic features and differentiate depositional facies that are difficult to distinguish with existing borehole imaging technologies.
The new high-definition OBM imaging technology was run in the well, achieving 80% circumferential coverage in the 9⅞-in. borehole and acquiring borehole images during down and up passes. As a result of reduced and less severe stick-and-slip events, the downlogged images were of noticeably higher quality.
Photorealistic formation images revealed greater structural complexity than expected, including unconformities, faults, and natural fractures. Subvertical drilling-induced fractures helped determine maximum horizontal-stress orientation. The geologists distinguished numerous depositional environments, including channel fill, lobe or sheet sands, and slump deposits, and were able to observe and directly measure frequent indicators of paleotransport direction.
Significant slumped sand sections were identifiable, based on texture and rapid changes in dip that conventional OBM images would have overlooked (Fig. 2). Interpretation of similar legacy images most likely would have mistaken these deposits for channel sands and included them in the net pay.
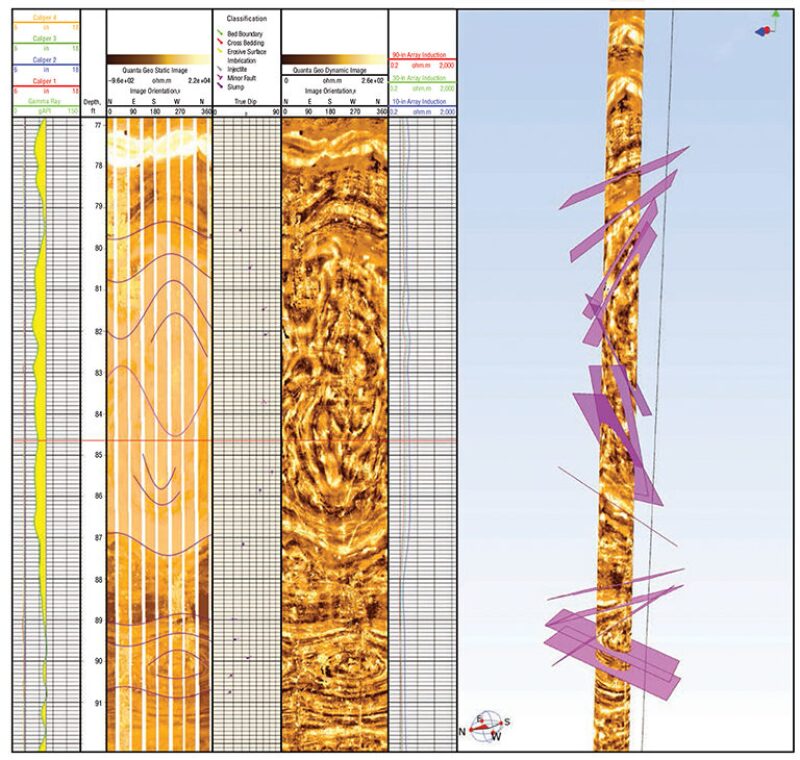
An operator in the Utica Shale drilled a vertical evaluation well using OBM and collected 85 sidewall core samples. To characterize natural fractures and precisely correlate the core plugs to the petrophysical logs, the operator ran the new photorealistic OBM imager and achieved a 93% circumferential coverage in the 8½-in. hole.
All core points were imaged, enabling analysts to match lab measurements with specific laminae and determine how well each core sample represented bulk reservoir properties. Subvertical fractures were clearly observed nearly parallel to the wellbore, indicating that the dominant fracture system strikes north-northwest to south-southwest at 80°–260°. As a result, the operator was able to calculate the opening pressure necessary to reactivate these fractures during hydraulic fracture stimulation.
Conclusion
Borehole imaging in OBM has finally caught up with, and in certain ways, surpassed the quality and coverage of imaging in WBM. Innovative tool design enables operators to minimize operational risks, eliminate unnecessary runs, and reduce rig time associated with logging borehole images. The new photorealistic images enable geoscientists to unravel complex structural and stratigraphic features, build more accurate reservoir models, improve well placement, and optimize completions in conventional and unconventional plays.