The significant temperature difference between the fractured and nonfractured regions during the stimulation-fluid flowback period can be useful for fracture diagnosis. Recent developments in downhole temperature-monitoring systems open new possibilities to detect these temperature variations to perform production-logging analyses. In the complete paper, the authors derive a novel analytical solution to model the temperature signal associated with the shut-in during flowback and production periods. The output of this work can contribute to production-logging, warmback, and wellbore-storage analyses to achieve successful fracture diagnostic.
Introduction
Information about individual fractures is critical in determining whether fracturing jobs have been successful or refracturing is required.
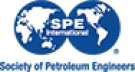