Asphaltenes represent the heaviest fraction of crude oil, which are known to precipitate when the crude is added to aliphatic solvents such as n-pentane or n-heptane and yet remain soluble in light aromatic solvents such as benzene or toluene (Gawrys et al. 2006; Borton et al. 2010). They are characterized by highly complex structures that contain multiple aromatic rings and have a large hetero-atom content (e.g., nitrogen, oxygen, and sulfur) and metal content (e.g., vanadium and nickel) (Yarranton 2000; Hashmi and Firoozabadi 2012).
Asphaltenes tend to self-associate on a molecular level, depending on the composition, temperature, and pressure of the system. Precipitation of the particles out of solution results in flocculation, where they begin to deposit on hydrophobic surfaces such as metal pipes and surface equipment used for the production and transportation of crude oils (Khvostichenko and Andersen 2009). These tendencies result in reduced flow or complete blockage of producing wells and surface equipment, including pumps, pipelines, and separators.
Currently, the only methods of treatment are through the use of chemical dispersants and inhibitors, which increase the stability of asphaltenes to prevent deposition. Once asphaltene deposition has occurred, running a “pig” through the pipeline is often the method used to scrape the solids that accumulated on the walls of the pipe. It is known that asphaltene molecules can be polarized, gaining an electric charge by introducing an electrostatic field (Hashmi and Firoozabadi 2012; Khvostichenko and Andersen 2009; Khvostichenko and Andersen 2010; Hosseini et al. 2016). The polarity of the asphaltene particles is directly related to its hetero-atom content, with a higher hetero-atom content giving increased levels of polarity and a higher rate of aggregation (Hosseini et al. 2016).
Experimental Device
Our ultimate goal is to build a device (Fig. 1a above) that would remove asphaltenes from crude oil near the point of production, using electrokinetics. Thus, a scaled-down device (Fig. 1b) was fabricated and tested using a model oil to prove the concept and study some of the parameters that would influence the design of a larger-scale device.
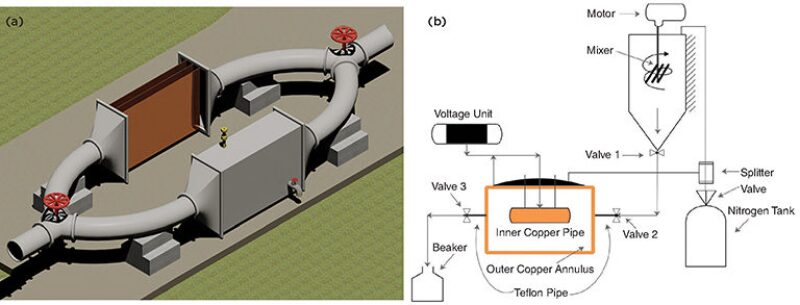
An annular chamber was conceived where model oil flowed through the annular space while applying a high voltage to create an electrostatic field so that the asphaltene electrodeposition under dynamic conditions could be investigated. A copper annulus was equipped as an electrode pair with a high-voltage power supply, and the model oil was gravity-fed into the charged annulus under varying flow rates. The model oil consisted of a 30:70 mixture of heptane and toluene by volume, called “heptol-70,” to which asphaltenes where added (2 wt%).
Saturates, aromatics, resins, and asphaltenes (SARA) are all components of crude oil that can be precipitated from it. Our earlier work applied electrokinetics under “static conditions” to the asphaltene fraction only, and concluded that the asphaltene particles could be attracted to the anode (Hosseini et al. 2016).
Work by Khvostichenko and Anderson (2010) indicated that resins may neutralize the asphaltene charge, affecting the electrodeposition of asphaltenes. To understand whether the other SARA components found in crude oil would interfere with the electrokinetic process under “dynamic conditions,” saturates, aromatics, and possibly resins were used along with the asphaltene particles in the heptol-70. While it is unlikely that the precipitates used in our study contained resins, because of the procedures that were used, we cannot rule out the possibility at this early stage of the work.
In addition, we conducted preliminary tests to compare two procedures for preparing the model oil, referred to as a “dissolution” or “precipitation” method (Khvostichenko and Andersen 2010). The dissolution approach involved adding the solid asphaltenes to the heptol, whereas the precipitation approach involved dissolving the solid asphaltenes in toluene and then adding heptane.
For the asphaltenes and heptol-70 used in our studies, our preliminary tests showed no measurable difference in the polarity of the particles when prepared using the two methods, and we used the precipitation method for all the dynamic tests.
The small-scale device consisted of a copper annulus and was charged to create an electric field; the inner pipe being charged negatively and the outer positively.
Testing
With the heptol-70 containing a 2-wt% concentration of asphaltenes in the elevated reservoir and the desired flow rate achieved, the power supply was turned on for 5 minutes. The average flow rate was measured while applying voltage. Once completed, the anode was removed and photographed, and the asphaltenes were scraped from the anode and collected so that the mass removed could be weighed.
Three flow rates were used while keeping the duration of the experimental run and the voltage constant at 5 minutes and 5,000 V, respectively. Figs. 2a through 2c show the deposition of asphaltene particles onto the inner copper pipe for the three flow rates. It is clear from the three figures that “the highest level of deposition” on the negative electrode corresponds with the highest flow rate, or “the shortest hydraulic retention time (HRT).” Fig. 2d shows the deposition layer being scraped from the anode, and the gravimetric data confirmed the qualitative results.
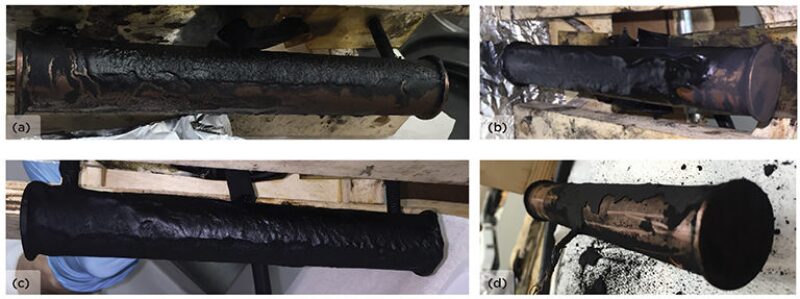
The dynamic tests were repeated and confirmed an inverse relationship between HRT and amount deposited onto the negative electrode—i.e., higher flow rates resulted in a higher degree of deposition. These results imply that the deposition of the asphaltenes is dependent upon the amount of particles that come in contact with the charged electrode, and also indicates that the efficiency of the electrokinetic process is high for the range of flow rates tested. We did observe some settlement of asphaltene particles at the bottom of the chamber that we attribute to the design of the device, where a small lip surrounding the exit acts to trap some of the particles.
Data Gathered
The data gathered at this early stage of research suggest that the particles, a mixture of asphaltenes, saturates, aromatics, and possibly resins, can be deposited onto an electrode and removed from the flow stream as a means of addressing a critical flow-assurance problem.
Future experiments are planned to explore the limitations of the process in terms of applied voltage and asphaltene loading. Our future research will include a wider variety of solvents/solvent properties with the ultimate goal of developing a means of predicting the effect on the asphaltene particles a priori. This will help to guide an overall design for our device before the scaleup to a prototype that will be applicable to produced crude. We are also considering a redesigned negative electrode to increase the surface area for the asphaltene particles to deposit.
The Reynolds number for these experimental conditions was above 106, indicating a turbulent flow regime within the charged annulus. We believe that turbulent flow is desirable because it would induce greater particle movement within the charged annulus, leading to more asphaltenes coming in close contact with the electrode, which would allow them to deposit onto it. However, we have not tested this assumption.
We noted that the experimental design could not avoid some settlement of particles because of physical trapping near the outlet of our annular device. As our studies are intended to examine the behavior of the particles only, we will strive to eliminate this phenomenon in our future experiments. However, for future industry applications, this phenomenon would help to remove more particles from the flow stream.
Acknowledgment
We would like to recognize Bionka Edmundson and Pushpesh Sharma for their help in the laboratory, and Professor Scott Randall for his guidance.
References
Borton D., Pinkston D., Hurt M.R. et al. 2010. Molecular Structures of Asphaltenes Based on the Dissociation Reactions of Their Ions in Mass Spectrometry. Energy & Fuels 24: 5548–5559.
Gawrys K.L., Blankenship G.A., Kilpatrick P.K. 2006. On the Distribution of Chemical Properties and Aggregation of Solubility Fractions in Asphaltenes. Energy & Fuels 20: 705–714.
Hashmi S. and Firoozabadi, A. 2012. Controlling Nonpolar Colloidal Asphaltene Aggregation by Electrostatic Repulsion. Energy & Fuels 26 (7): 4438–4444.
Hosseini A., Zare E., Ayatollahi S. et al. 2016. Experimental Study and Analysis of the Effects of Electrostatic Field on Asphaltene Kinetic Behavior. Fuel 178: 234–242.
Khvostichenko D. and Andersen S. 2009. Electrodeposition of Asphaltenes. Preliminary Studies on Electrodeposition from Oil-Heptane Mixtures. Energy & Fuels 23 (2): 811–819.
Khvostichenko D. and Andersen S. 2010. Electrodeposition of Asphaltenes. 2. Effect of Resins and Additives. Energy & Fuels 24: 2327–2336.
Yarranton A.H. 2000. Asphaltene Deposition. Presented at the Canadian International Petroleum Conference, Calgary, 4–8 June. PETSOC-2000-099-EA.