Exciting hydrocarbon discoveries of mind-bending quantities are being made in the far reaches of our solar system and our own Milky Way galaxy. A new paper (http://www.jpl.nasa.gov/news/news.php?release=2013-010) by scientists on NASA’s Cassini-Huygens mission finds that blocks of hydrocarbon ice might float upon the surface of existing lakes and seas of liquid methane and ethane on Saturn’s largest moon, Titan. Findings from a recent study (http://arxiv.org/pdf/1210.8178v1) of the Horsehead Nebula, part of the Orion Constellation, propose the discovery of a new interstellar molecule, the cation C3H+ (propynylidyne). C3H+ was one of 30 molecules identified in the region, including several small hydrocarbons.
Titan is about a billion miles away from Earth (1.5 light-hours) and the Horsehead Nebula is 1,300 light-years away (7.6 quadrillion miles). Why should the petroleum industry pay attention to the existence of hydrocarbons at impracticable distances from Earth?
Perhaps instead it would make more sense to focus on deep Earth.
However, there is a sense in which attempting to look below the surface of our own planet is a far more difficult task than exploring outer space: We can send a human 238,900 miles away to the surface of our moon, and humans routinely spend months on-board the International Space Station, which is maintained at an orbital altitude of between 205 and 255 miles above Earth.
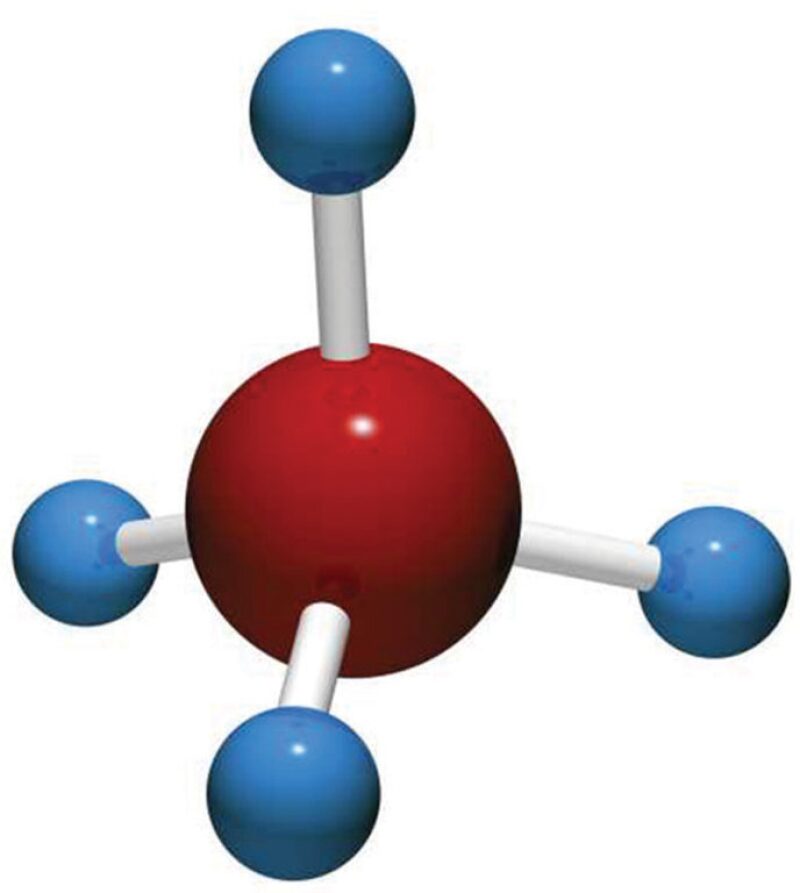
But only three humans have been to the Challenger Deep, first sounded during the HMS Challenger expedition (December 1872 to May 1876) at the southern end of the 1,580-mile-long, crescent-shaped Mariana Trench some 210 miles southwest of Guam, which at 36,069±131 ft (a little less than 7 miles) below sea level is the deepest point in Earth’s oceans. Jacques Piccard and Don Walsh spent only 20 minutes at a depth of 35,814 ft on 20 January 1960, in the bathyscaphe Trieste. Filmmaker James Cameron spent a little over 3 hours there on 25 March 2012, in his specialized submarine called the Deepsea Challenger.
A few deep-sea remotely operated vehicles have also plumbed the depths of the Challenger Deep.
Of course, for humans to actually enter the Earth’s crust is impossible without clearing and bolstering a mineshaft. The deepest humans have ever traveled into the Earth is at the TauTona gold mine, near Carletonville, South Africa, which extends some 2.4 miles underground.
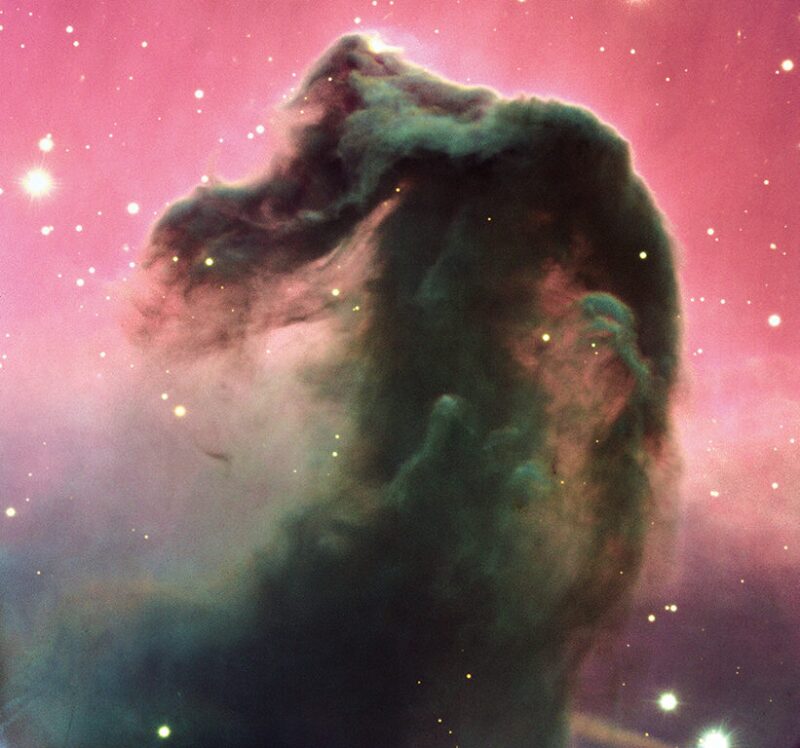
Exploring Earth’s Crust
If we can’t explore first-hand the Earth beneath our feet, what means have we developed to further our understanding of it? Earth’s interior—largely concentrating on the crust—is generally delineated through the following means, which all fall under the provenance of the oil and gas industry:
- From the surface by observation, such as of Earth’s topography, bathymetry, and rock outcroppings.
- From the surface by inference, such as with the use of seismic technology through bombardment with sound that travels as compression waves through the Earth, bouncing off the subsurface such that the returning waves can be interpreted as characterizing structures beneath Earth’s surface.
- Through combining drilling and inference with measurement- or logging-while-drilling sensors (or descending the borehole with wireline survey equipment following drilling), that incorporate computerized and miniaturized gyroscopes, magnetometers, calipers, and accelerometers to determine borehole inclination, toolface orientation, and azimuth; high-speed telemetry for quick downhole digital data transmission to surface; or natural gamma ray, nuclear magnetic resonance, and pulsed neutron generator technology for formation evaluation.
- Through direct evidence of subsurface composition through samples brought to surface from greater depths by earthquake, plate tectonic movement, and volcanic activity, deep mines, and through drilling and obtaining and analyzing coring samples. This latter technique is used for offshore scientific Earth exploration and in addition is widely used for greater precision in evaluating wells in the petroleum industry.
- Through experimentation and modeling with materials at pressures and temperatures characteristic of Earth’s subsurface. Not only are scientific hypotheses tested in this way, but this is an essential part of the oil and gas industry’s downhole-tool research, development, and testing, as well as modeling of hydrocarbon flow under geopressure.
Technological Development Synergies |
Further interplay between deep space and Earth’s petroleum industry may lie in synergies to be found in technological development. Rice Space Institute director and professor of physics and astronomy David Alexander comments about space travel, “We’re trying to put humans into an environment that doesn’t want them.” From this point of view, he says, “The oil and gas and space industries have a lot of problems in common.” “I don’t think people fully appreciate one of NASA’s main goals in human space flight,” says Alexander. “That is: They don’t want a single astronaut to die. They want them all to come home healthy.” With both industries’ risk aversion and emphasis on safety processes, not only would there be an overlap in health, safety, security, and environment issues, but also technology must be developed that is rugged, precision-built with redundant systems for continuous operation and reliability, and capable of withstanding and operating faultlessly in harsh environments over long periods of time. The 2012 paper OTC 22989, “Space Robotics Technologies for Deep Well Operations,” by H. Nayar, et al. of the Jet Propulsion Laboratory (JPL), California Institute of Technology, highlights the similarities between space applications and applications in the oil and gas industry. “In both cases, systems are deployed at remote locations with limited access for intervention, maintenance, or repair. The operational environments are often hostile with harsh temperatures and pressures and corrosive materials. Reliability of systems is extremely important in space and in the oil and gas industry. Operations in the respective environments have high risk and the capabilities for replicating environmental conditions for testing and failure mitigation are limited. Failures can be catastrophic and the cost, financially and in public perception, can erode support for programs. Due to these similarities, there is much that the space and energy sectors can learn and benefit from each other.” The authors point out that “JPL’s design of science instruments and sensors in unique planetary surface environments enables translation of engineering heritage to capabilities necessary for extreme terrestrial environments.” They give autonomous underwater vehicles (AUVs) as an example of a type of technology for which JPL’s capabilities would be appropriate, citing JPL’s experience with remotely operated vehicles (ROVs) “examining exploration of saline water oceans on Europa, Enceledus, or hydrocarbon lakes on Titan.” With JPL’s experience in simulating vehicles driving on the surface of Mars and the moon, as well as of the entry, descent, and landing of spacecraft on Mars, the authors state, “Modeling and understanding of dynamic phenomena in the oil and gas industry could help in understanding and predicting their behavior and in developing techniques for influencing or controlling them. Flow of material through permeable media, drilling, excavating and other types of terrain interaction, and operations in terrestrial surface and underwater, surface, and seabed marine application are potential areas where the technologies we have described could be used.” JPL’s Mars rovers might also be useful to the industry. “The Axel rovers can be repurposed to provide access to a large variety of terrain types in the oil and gas industry that are either dangerous, inaccessible, or costly to reach with human workers.” The authors also identify visual odometry, “which has been used on the Mars Exploration Rovers and on research rovers to accurately determine vehicle motion without the need for GPS. It can be used in the energy industry to precisely measure the motion of moving platforms, such as ROVs, AUVs, or even instrumented drill bits, which also would not have access to GPS data.” Oceaneering, founded in 1964, has grown from an air and mixed-gas diving business in the Gulf of Mexico to a global oilfield provider of engineered services primarily to the offshore oil and gas industry, with a focus on deepwater applications. A section of the business, called Oceaneering Space Systems (OSS), develops a wide range of products for use in the aerospace industry. For example, the Robotics & Automation group specializes in creating custom automated and remotely controlled systems for use on Earth or in space, including the International Space Station (ISS), formerly for the Space Shuttle, and for commercial space enterprises. In another example, in March 2009, OSS was selected by NASA to develop and produce the Constellation Space Suite System (CSSS), a new space suit for solar system exploration. The CSSS is a key component of extravehicular activity (EVA or spacewalking) system for NASA’s Space Exploration program. It will be used to sustain the US presence in low Earth orbit, help establish an outpost on the moon, and lay the foundation for further human exploration of the solar system. In further examples, since 1978, OSS has developed and delivered thousands of end items to NASA and other contractors for intravehicular (IV) or EVA. OSS has developed a number of advanced life support systems and technologies applicable to future spacesuits and spacecraft, and full suites of tools and equipment for assembly, maintenance, and repair of satellites and other spacecraft, including the ISS. OSS is responsible for the sustaining engineering and replenishment of all operational EVA tools and crew aids, and for the operations of NASA’s Sonny Carter Training Facility Neutral Buoyancy Lab, NASA’s primary training center for EVA. Rice Space Institute’s Alexander also pointed to a Planetary Drilling Workshop, held 29 August 2011 in Stavanger, Norway, which brought together participants from several Norwegian space companies and agencies, as well as members of the oil and gas industry. Several topics were discussed, including planetary exploration and drilling, Mars and asteroids, and new generation drilling, which encompassed automation, simplicity and flexibility, long distance, rig-less exploration, drilling autonomy, and extreme sensors. The 40 participants spent the afternoon working on one of three creative tasks: To save an asteroid from colliding with Earth by drilling it, placing a nuclear bomb in it, and blowing it up; drilling on the moon for exploitation of the lunar polar ice and exploring so-called “lunar skylights”; drilling on Mars through its regolith (permafrost) to ground ice table (1 km), to liquid H2O (2 km) to sense organisms, through ice to rock (2 km), and deeper (5 to 10 km) through regolith to heat. |
Deep Scientific Ocean Drilling and the Offshore Petroleum Industry
Understanding Earth’s crust has mostly centered on exploring where it is thinnest and therefore closest to Earth’s mantle—the ocean deep—where the crust is seldom more than 3.1 miles thick. Scientific exploration of the ocean crust in some ways has pushed well beyond the limits encountered in offshore oil and gas exploration and production (E&P), presaging a future in which E&P might take place at ocean locations and depths hitherto beyond the industry’s scope.
Using technologies developed for the offshore upstream oil and gas industry, the Integrated Ocean Drilling Program (IODP), which undertook its first expedition in 2004, just completed its 45th on 12 February 2013. The expeditions’ purpose is to gain a greater understanding of the Earth’s crust through drilling for and analyzing cores, approximately 60,000 of which were obtained between 1968 and 2013.
The IODP, formed in 2003, distinguishes itself from its legacy programs by employing multiple drilling platforms—the JOIDES Resolution, the Chikyu, and Mission-Specific Platforms. Its predecessor organizations—the Deep Sea Drilling Project (DSDP), which began in 1966, and the Ocean Drilling Program (ODP), which began in 1985—conducted close to 200 scientific ocean drilling expeditions. A global map of scientific ocean drilling sites, from ODP, DSDP, and IODP expeditions, displays hundreds of dots peppering the world’s oceans.
A new international research program—called the International Ocean Discovery Program—will start in October 2013, with the support of 26 nations. Drawing on information from beneath the ocean floor, brought to the surface by ocean coring techniques and borehole observatories, the program will continue the quest to bring together the Earth, ocean, atmospheric, and life sciences with the common goal of understanding Earth’s past, present, and future.
It all began in 1961, when offshore drilling technology was successfully used to recover the first sample of oceanic crust by the CUSS 1, the first drillship to be equipped with dynamic positioning. In API Conference Paper 58-124, “A Method of Drilling from a Floating Vessel and the CUSS 1,” by R.F. Bauer, A.J. Field, and H. Stratton of Union Oil of California, the authors state “Several years of research led ultimately to the design and construction of the floating drilling barge CUSS 1.” They identified the CUSS group as Continental, Union, Shell, and Superior Oil Companies.
This initial quest to explore oceanic crust was known as Project Mohole, an ambitious attempt to drill through Earth’s crust into the Mohorovičić discontinuity. The boundary between Earth’s crust and mantle, the Mohorovičić discontinuity is 3 to 6 miles below the ocean floor and 10 to 60 miles beneath typical continents. Project Mohole drilled a total of five holes in 11,700 ft of water, one of which extended to 601 ft—hardly the 4.35 miles needed to reach its destination. But it was a start, and thus scientific drilling took root as a new scientific pursuit.
The project’s 11,700-ft water depth is also significant. It is only in recent years that the oil and gas industry has drilled at depths approaching that. According to Starr Spencer, writing for Platt’s The Barrel (20 July 2012) about current oil and gas industry trends, “The newest ultra-deepwater rigs coming into the market these days are equipped to drill in 10,000 ft of water, and can gear up for waters 12,000 ft deep with some added equipment. The most state-of-the-art rigs can also drill 40,000 ft below the mud line. That is well past current needs; the deepest well ever drilled so far in the US Gulf, for example, lies in about 10,000 ft of water and the deepest total depth has been about 35,000 ft.”
In the August 1975 issue of the Journal of Petroleum Technology, V.F. Larson, an SPE member as well as participant in the DSDP, wrote, in “Deep-Water Coring for Scientific Purposes,” “Since 1968, the Deep Sea Drilling Project (DSDP) has used a dynamically positioned drillship, the Glomar Challenger, in a world-wide scientific research program for recovering sediment cores from the deep oceans and adjacent seas. Short sections of underlying igneous and metamorphic rocks also have been recovered. The objective of this basic geologic research is to learn about the origin and history of the Earth through the study of samples obtained in previously inaccessible sites. Operations have been conducted successfully in water depths greater than 20,000 ft and in polar latitudes to 77° south and 76° north. More than 120,000 ft of cores have been recovered.”
He also noted further, “To minimize cost, proven oilwell drilling equipment was used wherever possible.”
Deep Scientific Drilling of Earth’s Crust on Land
None of the offshore scientific drilling expeditions penetrated the Earth’s crust as deep as the Kola Superdeep Drill Hole. Beginning mid-1970 and ending in 1989, a 40,226-ft borehole was drilled on the Kola Peninsula in what was then known as the Union of Soviet Socialist Republics (USSR) and is now within Russia. In 1986, the US Geological Survey (USGS) printed a detailed summary (http://pubs.usgs.gov/of/1986/0517/report.pdf) of a book, titled The Kola Superdeep Drill Hole, written by Ye. A. Kozlovskiy in Russian in 1984 of the project’s findings to that point. The book covered extensive details of the general geology, rocks and rock-forming minerals, geochemistry, mineralization, gases, organic material, hydrogeology, geophysics, magnetic properties, well design, and drilling.
From the introduction to this book, it appears the motivation for drilling the hole was largely political, with “attention to strengthening the mineral resource base of the country and to improving exploration methods.” One of the book’s opening statements is: “The importance of mineral resources in the modern world increases constantly. Oil, gas, and atomic raw materials are required more and more as a source of energy.” From this, it would appear that the oil and gas industry had at least as much to gain from this project as scientists in their understanding of Earth’s crust.
The oil and gas industry, although it has never drilled deeper than Kola’s vertical depth, has drilled two extended-reach boreholes that penetrated greater measured depths: the 40,318-ft Al Shaheen well in Qatar, drilled by Transocean for Maersk in 2008; and the 40,502-ft Sakhalin-1 Odoptu OP-11 well offshore the Russian island of Sakhalin, drilled by operator Exxon Neftegas in 2011.
The Deep Carbon Observatory and Hydrocarbons’ Origins
For the reasons stated earlier—namely that exploring beneath Earth’s surface is highly limited as well as expensive and logistically challenging—scientists’ understanding of the depths of our own planet is hindered by what physicist Thomas Gold, in The Deep Hot Biosphere, refers to “a sort of ‘surface chauvinism.’” The Deep Carbon Observatory (DCO) seeks to get past that bias.
The DCO is an international, cross-disciplinary organization, founded in 2009, whose stated vision is “Achieving transformational understanding of carbon’s chemical and biological roles in Earth’s interior.” One of its four directorates is devoted to “Deep Energy,” and several meetings and workshops have been held since 2009, some of which delved specifically into the origin and role of hydrocarbons in deep Earth composition and material properties. The Deep Energy Directorate is dedicated to developing a fundamental understanding of environments and processes—from the molecular to the global scale—that regulate the volume and rates of production and transformation of hydrocarbons and other organic species in the crust and mantle through geological time. Members of the DCO have an interest in deepening humanity’s understanding of Earth’s geophysics and the implications this might have in understanding issues that affect the petroleum industry, such as carbon capture and sequestration and the identification of hydrocarbon resources into the deep future.
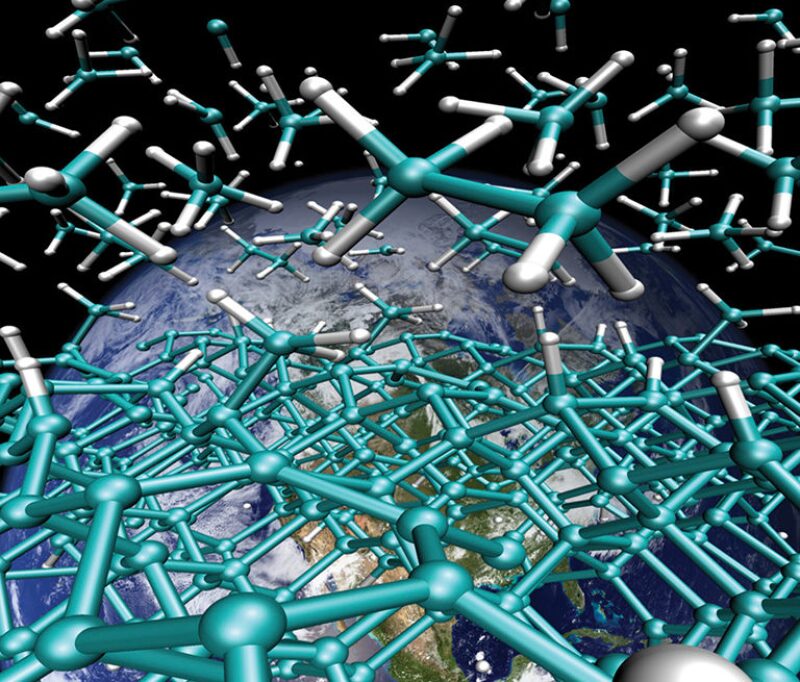
“It is generally accepted that the origin of methane and related light hydrocarbons found in surface and near-surface reservoirs is due to one of two mechanistic pathways: microbial-based digestion of organic matter or by thermal degradation of organic compounds,” states a white paper that was an outcome of a DCO Energy, Environment, and Climate workshop, co-chaired by Detlef Hohl of Shell Projects & Technology and Christian Mailhiot of Lawrence Livermore National Laboratory, whose 34 participant scientists from academia, industry, and government met in July 2010. “This long-held view has been challenged by reports of high-temperature methane-rich fluids venting from sediment-poor mid-ocean ridges, hydrocarbon seepages from terrestrial regions dominated by ultramafic rocks, methane-bearing fluids from Precambrian shields, and fluid inclusions in mantle and igneous rocks.”
The view that hydrocarbons may have abiogenic as well as biogenic origins is a controversy reaching back almost to the first major oil gusher, Edwin Drake’s Titusville, Pennsylvania, well that in 1859 ushered in the modern hydrocarbon-driven era. Dmitri Mendeleev (1834–1907), Russian chemist, inventor, and creator of the first version of the Periodic Table of Elements, was among the early advocates of a nonbiological origin of petroleum. He wrote a paper to that effect in 1877, in which he stated, “The capital fact to note is that petroleum was born in the depths of the Earth, and that it is only there that we must seek its origin.”
The subject was alive and well in respected scientific circles more than a century later. In the introduction to the 1993 USGS Professional Paper 1570, The Future of Energy Gases, authors D.G. Howell, F. Cole, M. Fanelli, and K. Wiese state, “Most of the methane that is extracted from the large natural-gas accumulations found in the Earth’s crust is probably either biogenic or thermogenic in origin. The role of abiogenic methane in the formation of gas accumulation is a controversial issue.”
In another chapter within USGS 1570, titled “Abiogenic Hydrocarbons and Mantle Helium in Oil and Gas Fields,” authors P.D. Jenden (Chevron), D.R. Hilton, I.R. Kaplan, and H. Craig write “The chemistry of the Earth’s interior is poorly understood … and methane, rather than carbon dioxide, may be the dominant form of carbon throughout much of the mantle.” They state further, “The thermodynamic stability of methane in the Earth’s mantle is a critical, unresolved issue.”
The thermodynamic stability issue is addressed via experimental simulations discussed in the paper “Stability of Hydrocarbons at Deep Earth Pressures and Temperatures,” by L. Spanu, D. Donadio, D. Hohl (Shell), E. Schwegler, and G. Galli, published in the 18 March 2011 Proceedings of the National Academy of Sciences of the USA. The authors state, “Our results indicate that the formation of higher hydrocarbons from pure methane is likely to occur when carbon-bearing fluids are in contact with metals [e.g., iridium] or solid carbon phases [e.g., diamond surfaces and graphite]. On the other hand, the extent of free elemental carbon at depth in the Earth is not known.”
An earlier paper—“Generation of Methane in the Earth’s Mantle: In Situ High Pressure–Temperature Measurements of Carbonate Reduction,” by H.P. Scott, R.J. Hemley, H. Mao, D.R. Herschbach, L.E. Fried, W.M. Howard, and S. Bastea, published in the 28 September 2004 Proceedings of the National Academy of Sciences of the USA—describes very high pressure–temperature experiments that demonstrated the formation of hydrocarbons from the reduction of carbonate, an abiogenic mechanism.
How do experiments like this affect the oil and gas industry’s ongoing quest for further resources? USGS emeritus organic geochemist Keith Kvenvolden, a pioneer in methane hydrate research, says, “In the petroleum industry, people explore by having a certain model in mind—biotic oil is that model. They’ve figured it out, that’s why they’re so successful.” He cautions, “Deep energy doesn’t necessarily mean abiotic oil.”
However, the DCO white paper asserts “that our current understanding of thermogenic and biogenic natural gas generation and transport cannot and does not explain the chemical and isotropic composition of some major gas reservoirs.” It cites the super-giant Urengoy gas field in western Siberia and fields in the Songliao basin, China, as among the reservoirs whose origins challenge current understanding. “Both natural gas systems are in proximity to geological terrain favorable to either abiogenic hydrocarbon production routes or direct mantle fluid input and have been proposed as a possible source of the anomalous gas field compositions.”
Understanding of such reservoirs may lead to future searches for resources in areas currently not under investigation. In fact, notes DCO Deep Energy chair David Cole, “One of the goals of the DCO is to gain access to these types of systems to address testable hypotheses that focus on the origin of the hydrocarbons. The goal is to do this through the use of new analytical instruments, novel simulation experiments, and modeling.”
Further Benefits of the Deep Carbon Observatory (DCO) |
The energy industry in particular will be a prime beneficiary of the DCO program in terms of sustainable exploration and production of hydrocarbons, improved carbon capture and sequestration technologies, as well as other energy-related spin-offs.
|
—From a white paper that was an outcome of a DCO Energy, Environment, and Climate workshop, co-chaired by Detlef Hohl of Shell Projects & Technology and Christian Mailhiot of Lawrence Livermore National Laboratory, whose 34 participant scientists from academia, industry, and government met in July 2010. |
Note: The DCO Energy, Environment, and Climate Directorate evolved into the current DCO Deep Energy Directorate. “One of the goals of the DCO and Deep Energy in particular is to develop a fundamental understanding of the processes that may lead to the formation of hydrocarbons in the crust and mantle,” states DCO Deep Energy chair David Cole, professor, School of Earth Sciences at Ohio State University. “We do this,” he continues, “through a synergistic combination of field studies of representative geological systems (e.g., deep basins, Precambrian cratons, oceanic and continental hydrothermal systems, and nonvolcanic active tectonic regimes), bench-scale experiments that emulate deep Earth conditions, and modeling that spans over 14 orders of magnitude in length scale (nanometer to kilometer). These are underpinned in many cases through the implementation of new analytical, experimental, and computational techniques. An overarching goal will be to integrate all that we learn about deep carbon into robust global carbon cycle models.” Cole notes that this month (March), the DCO will release its first major collective publication, Carbon in Earth, which will appear as a volume in Reviews in Mineralogy and Geochemistry (published jointly by the Mineralogical Society of America and the Geochemical Society). |
Gas Hydrates: “Fire in the Ice” in Outer Space and Earth
“The gas hydrates, which are clathrate compounds of gases in a (cubic) ice matrix, are not known to occur naturally on Earth because of the unfavorable combination of temperatures, pressures, and gases that are poor hydrate formers in the Earth’s atmosphere,” writes Stanley L. Miller of the Department of Chemistry at University of California (San Diego), La Jolla, in “The Occurrence of Gas Hydrates in the Solar System,” 29 October 1961 Proceedings of the National Academy of Sciences of the USA.
He instead points toward outer space for their existence: “The factors which prevent the natural occurrence of the gas hydrates on the Earth do not apply to other parts of the solar system, and … these hydrates may play a significant role in the chemistry of the solar system.”
Prefiguring the Cassini-Huygens probe’s recent identification on Saturn’s moon Titan of vast quantities of liquid and hydrate methane and ethane, Miller writes, “There seems little doubt that the water on Titan occurs as methane hydrate rather than ice. Since methane did not escape before this satellite was formed, the same is probably true for the other satellites of Saturn and Jupiter.”
He surmises that, “Although most of the methane has escaped from the atmosphere of these other satellites, the methane in the hydrate of the interior would diffuse slowly to the surface.”
“Therefore,” he writes, “it seems likely that a large fraction of the mass of these satellites is methane hydrate.”
Miller further asserts, “It is possible that some of the interstellar dust is gas hydrate,” and concludes, “it would appear likely that gas hydrates are present in several parts of the solar system” and “gas hydrates appear to have played a significant role in the chemistry of planet formation.”
Although in fact scientific research into the nature of methane hydrates dates back to the early 1800s, such experiments involved its synthesis under laboratory conditions. Until the 1930s, when hydrates were observed forming in natural-gas pipelines—actually a nuisance that sometimes blocked the flow of gas—methane hydrates were still not expected to occur in the natural world on Earth.
Small amounts of methane hydrates were encountered in the world under natural conditions before the early 1980s. In the 1960s, they were found in subsurface sediments of the Messoyahka gas field of the western Siberia basin; in the 1970s, in drilling in the MacKenzie Delta; and in 1980, in drilling along the Middle America Trench.
Early in the 1980s, Thomas H. Shipley and Borys M. Didyk state in “Occurrence of Methane Hydrates Offshore Southern Mexico” (Initial Reports of the DSDP, Volume 66), “The recognition of natural gas hydrates is increasing rapidly and has been recently reviewed by Kvenvolden and Menamin (1980)” in USGS Circular No. 825: “Hydrates of Natural Gas: A Review of Their Geologic Occurrence.”
Shipley and Didyk discuss Deep Sea Drilling Project Leg 66 on which in 1981 “the first quantitative evidence for naturally occurring deep sea gas hydrates” was encountered during drilling on the Mexican continental margin southwest of Acapulco at three different drill sites.
They point out the significance of the discovery: “Naturally occurring methane hydrate is an interesting substance because it may contain up to 170 ml of methane per milliliter of interstitial water. Also, completely gas-hydrated sediments might result in reduced permeability and permit gas and oil accumulation below a hydrate cap. Thus, knowledge of these substances and their possible presence in marine sediments is important for evaluating their potential as an energy resource and for drilling safety and pollution prevention.”
The presence of gas hydrates on Earth became not only widely known, but the issue of their origin was also probed. In 1982, SPE members Walter Rose of the Institute of Gas Technology and Hans Olaf Pfannkuch of the University of Minnesota wrote in paper SPE/DOE 10836, “Unconventional Ideas About Unconventional Gas”: “Vast quantities of natural gas exist as solid hydrates in the world’s permafrost and deep sea environments. … Their ubiquity provides an interesting link to another unconventional hypothesis of unconventional gas supply, namely the abiogenic origin of deep Earth gas.”
By 2012, significant amounts of gas hydrate research had taken place, particularly through consortia of government, industry, and academic experts in the US, Europe, Japan, Canada, India, Korea, China, and Malaysia—and also by the United Nations. Two major research centers developed: The Center for Hydrate Research, which resides in the Chemical Engineering Department at the Colorado School of Mines; and the Centre for Gas Hydrate Research, based at the Institute for Petroleum Engineering at Heriot-Watt University, Edinburgh, Scotland.
A 2011 US Department of Energy National Energy Technology Laboratory (NETL) report titled Energy Resource Potential of Methane Hydrate, states “The worldwide volume of methane held in methane hydrates is immense and poorly known. Estimates range from 100,000 to more than 1,000,000 Tcf of natural gas.” This is known as “gas-in-place” (GIP). However, the GIP number is not meaningful for resource-based studies. The NETL report cautions, “only a small portion of this enormous resource is likely to be harvested as an energy fuel.”
Carolyn Ruppel, member of the USGS Gas Hydrates Project, writes in Methane Hydrates and the Future of Natural Gas, a supplement to a 2011 Massachusetts Institute of Technology Energy Institute (MITEI) report on natural gas, “It is important to note that there are as yet no proved reserves of gas hydrate since gas has never been produced from gas hydrate for more than a few days in research tests.”
“Given the overall uncertainty still associated with gas hydrates as a potential resource,” Ruppel says elsewhere in the supplement, “they have not been included in the Emissions Prediction and Policy Analysis (EPPA) model in MITEI’s Future of Natural Gas report. Still, gas hydrates remain a potentially large methane resource and must necessarily be included in any consideration of the natural gas supply beyond two decades from now.”
Hydrocarbons Proliferate in Space, Yet We’re Rooted on Earth
The presence of hydrocarbons—particularly methane hydrates—in space may yet yield insight into their presence on Earth. “We have not actively sought hydrocarbons elsewhere in the planetary system and the galaxy,” says David Alexander, professor of physics and astronomy and director of the Rice Space Institute. “But they are there. And they play a role in understanding the universe.” When and if hydrocarbons such as methane clathrates become serious objects of petroleum industry exploration and production, astrophysicists’ explanations of their presence elsewhere in the solar system and galaxy may play a role in identifying and safely exploiting them for human consumption.
Also, the question of determining whether the methane on Earth is biogenic or abiogenic in origin may at some point prove useful. Physicist Thomas Gold wrote “The Origin of Methane in the Crust of the Earth” in the 1993 USGS Professional Paper 1570, The Future of Energy Gases. “Hydrocarbons in our planetary system are certainly very abundant, and in all the extraterrestrial examples mentioned [in his paper] they are almost certainly not related to biology,” Gold states. “Also, hydrocarbons are prominent among the gases identified in the molecular clouds of the galaxy, and it is from such clouds that the solar system formed initially.”
He concludes, “If the main supply of the commercial quantities of hydrocarbons, both oil and gas, is indeed derived from mantle depths and from materials that were incorporated in the Earth at its formation, then many points in petroleum geology and in other aspects of geology will have to be reconsidered. The quantity and locations of gas and oil that can be found, the method of prospecting for them, and the technology involved will all be affected.”
A key question is: When will petroleum geologists find the economic need to change or expand their longstanding biogenic models for reservoir location and hydrocarbon flow? Based on those models, despite increasing consumption, proved reserves of hydrocarbons continue to climb. According to Energy Information Administration statistics, in 1980, world proved reserves of crude oil (Fig. 1) were 641.9 billion bbl. In 1990, they had climbed to 1000.3 billion bbl. By 2000, they were slightly more, at 1017.0 billion bbl. By 2010, crude reserves had climbed further to 1358.3 billion bbl. World consumption is projected to grow from 2005’s 84.2 million B/D to 2035’s 112.2 million B/D.
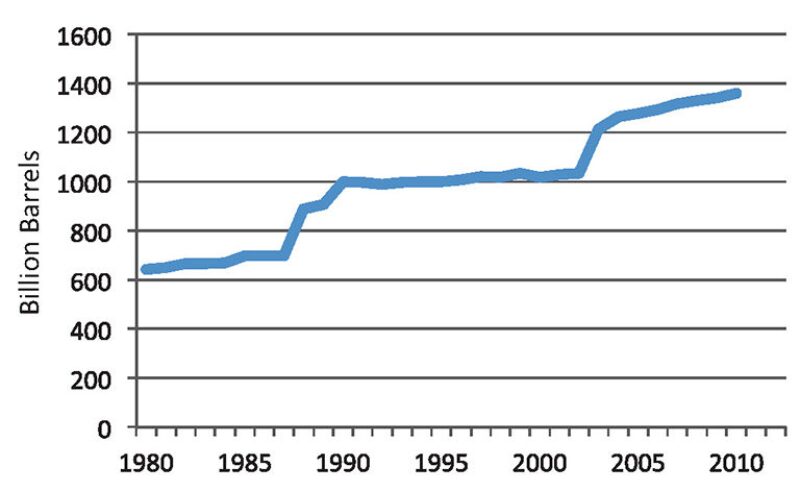
A similar trend exists for reserves and consumption of natural gas. In 1981, world proved reserves (Fig. 2) stood at 2629.0 Tcf. This climbed to 3965.5 Tcf by 1990 and 5159.6 Tcf by 2000. In 2010, the figure was 6669.3 Tcf. Annual world consumption of natural gas is projected to grow from 2005’s 101.8 Tcf to 2035’s 168.7 Tcf.
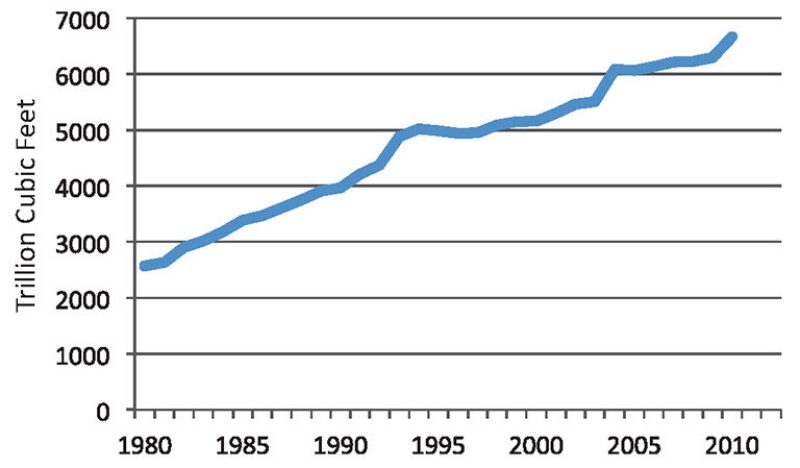
The keys to reserve replacement, however, are not entirely dependent on identifying new fields. One key is the convergence between higher oil and gas prices and the reclassification of previously known but uneconomic resources, moving them by means of the use of more expensive extractive technologies into the realm of proved, and thus economical, reserves. Higher oil and gas prices, too, permit more research and development expenditures, which tend to translate further into technology that enables not only more primary recovery, but also better secondary and tertiary recovery. Previously unexploited formations within previously exploited fields become economic.
A Glimpse Into the Future
Keeping alive the interplay between advances in understanding deep Earth, deep space, and the needs of the petroleum industry may lead us to a future shaped more by the intersection of these pursuits.
Who knows? Humanity’s deep future may lie on one or more of the 2,740 planet candidates identified up to early January 2013 by NASA’s Kepler mission. Launched 6 March 2009, NASA sent its first planet-hunting spacecraft on a 3-and-a-half-year mission—extended to 2016—to seek signs of other Earth-like planets. Kepler is specifically designed to survey our region of the Milky Way galaxy to discover hundreds of Earth-size and smaller planets in or near the habitable zone and determine the fraction of the hundreds of billions of stars in our galaxy that might have such planets.
One of the four newly identified super Earth-size planet candidates, KOI-172.02, orbits in the habitable zone of a star similar to our sun. The possible planet is approximately 1.5 times the radius of Earth and orbits its host star every 242 days.
However, the stars Kepler is observing are in the range of a few hundred to a few thousand light-years away. At 6 trillion miles per light-year, that represents travel that would occur over many generations.
And who knows how those space travelers would generate energy once they arrived at their destination? Would the planet have crude oil and natural gas? Or would we have graduated to the next generation of energy source by then?
Mining Insight |
Humanity’s understanding of deep space and deep Earth has grown since the first major gusher flowed in Titusville, Pennsylvania, in 1859. The following are some of the ways the petroleum industry has participated in this pursuit of knowledge: Through both developing and using technology originally meant to further the quest to penetrate the mysteries of space and delineate Earth’s crust beneath the ocean floor. Through investigating the nature of possible parallels between hydrocarbon presence on Earth and on other planetary or celestial objects. Through keeping open the question of whether there are abiogenic sources of hydrocarbons within Earth, particularly of methane. Through maintaining a voice in the conversation about the role of hydrocarbons in Earth’s overall carbon cycle. Through participating in challenging the conventional concept of the carbon cycle by instead seeing the cycle as emanating from and extending farther into deep Earth than has commonly been held. |