The pipelines and vast infrastructure required for oil and gas production and transport are constructed largely of carbon steel, which is highly susceptible to damage and failure as a result of direct or indirect microbially influenced corrosion (MIC). One approach to reduce or remediate MIC is by applying microbicides to affected assets such as storage tanks, pipelines, heat exchangers, and pumps. The objective of this paper is to generate efficacy data for biocidal formulations against corrosion-associated biofilms grown under anoxic and flowing conditions. The data are difficult to obtain because of the distinct nature of biofilms and growth conditions. It can be more representative when mimicking petroleum- and water-transporting pipelines.
To facilitate testing and comparison of products that successfully attenuate MIC under field-relevant conditions, a method was developed that allows consistent corrosion measurements to be made for corroding biofilms grown on steel surfaces under flowing and anoxic conditions.
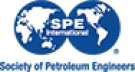