To maximize well productivity and reservoir oil recovery in mature fields, advanced lateral designs with intelligent completions have been implemented during the last decade. This paper describes an all-in-one system that combines nodal-analysis and numerical-simulation models to calculate the effect of intelligent-completion components—such as swell packers, internal control valves (ICVs), and inflow-control devices (ICDs)—on lateral production profiles. A 3D-grid, multiphase-flow, nonisothermal reservoir numerical simulator was used for advanced well design, and a wellbore nodal-analysis simulator was used to solve the partial-differential equations between the porous medium and the wellbore.
Introduction
Multilateral wells are often the best choice to maximize reserves recovery and contact with the reservoir and to extend the drainage area. However, several studies have revealed that multilateral wells exhibit a series of natural issues (e.g., high influx at lateral heels because of pressure drops). As a result of such issues, increased water or gas production can decrease oil production over time. Use of intelligent-valve technology can mitigate these problems significantly.
Reservoir Complexity and Heterogeneity
The reservoir model used here is a synthetic reservoir with an almost constant porosity of 20% and significant areal permeability variation from 1 to 500 md. The main depth is 8,000-ft true vertical depth subsurface. The net pay is 100 ft, and net/gross ratio used for the simulation is 0.8. A 3D sector numerical-simulation model was built with the following dimensions: x=100 cells, y=50 cells, and five layers, for a total of 25,000 cells.
The areal permeability distribution is taken from a geostatistical model. The permeability distribution is constrained by spatial carbonate-facies distribution honoring log and core data of injector and producer wells in the sector model. Reservoir-quality index (RQI) is calculated to screen areas with best reservoir quality; an area where RQI equals 10 is considered the best area to place multilaterals.
However, the reservoir exhibits strong permeability contrast, not only among layers but also inside the carbonate body, as shown in Fig. 1.
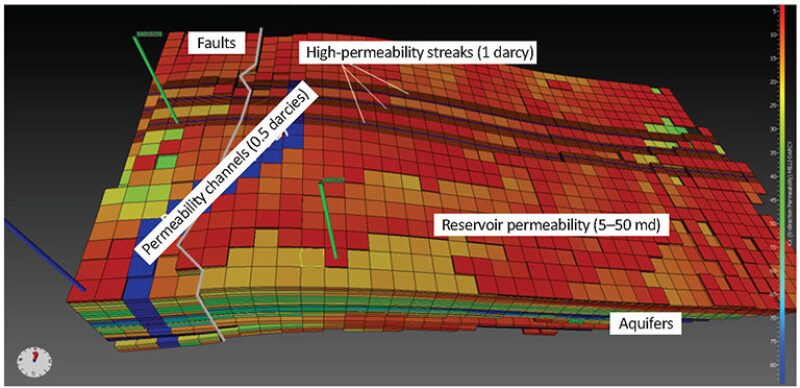
Multilateral-Well Completion
Two types of completions were selected to evaluate the coupling of the numerical and completion models: (1) openhole and (2) openhole completed with blank liners, swell packers, ICVs, and ICDs. The completion simulator evaluates the influx, pressure drop, and water-cut performance along the horizontal section of each lateral.
The openhole option has a drilled section of 2,000 ft and hole diameter of 6 in. The intelligent-completion option was designed with six segments, separated with swell packers. An optimization study determined that the optimal number of ICDs is 25, spaced at 80 ft over 1,800 ft.
Simulation Tools and Applications
Steady-State (SS) Completion-Simulation Model. The authors used a completion simulator that calculates the hydrodynamic behavior of the full lateral section. The completion design—with ICD valves, packer locations, gravel pack, ICV, and other completion components—is set up and designed in the SS model. The tool analyzes the effect of different completion schemes providing better results for oil rate and flowing bottomhole pressure. The tool can analyze the velocity, rate, and pressure drop across the reservoir, annulus, valves, tubing, joints, junctions, and wellhead. From numerical simulation, input and output files are downloaded into the SS model. Therefore, static and dynamic properties—such as cell porosity, permeability, thickness, cell reservoir pressure, and water saturation—are visualized in the model.
Numerical-Reservoir-Simulation Model. For forecasting and calculation of estimated ultimate recovery, the SS tool is coupled numerically with a numerical simulator that predicts water encroachment and oil-production performance at transient time. The numerical tool is a 3D-gridding, black-oil, pressure/volume/temperature-model, nonisothermal simulator that captures with high precision the effect of near-wellbore stimulations (such as hydraulic fractures), conformance, and special wellbore designs such as multilateral trajectories.
Coupling Reservoir and Well-Completion Simulators. Basically, the multilateral-well trajectory is designed and analyzed in the SS model. When the analysis is finished, the results are exported to the numerical model as the main trajectory.
The SS simulator handles the completion effect inside the wellbore architecture; the numerical simulation is used to optimize the completion design, even intelligent completions, to evaluate the completion efficiency and production performance over time. The reservoir simulator numerically solves the partial-differential equations between the porous medium and the wellbore. When modeling the effect of any of these completion components (e.g., ICDs, ICVs, packers), the hydraulic behavior between the annulus and tubing or downhole valve and tubing will respond in different ways, so water or gas reservoir performance across time will be optimized.
Multilateral Case Configuration
This study used an intelligent multilateral design with a cemented junction. The lateral wellbore is cemented for the first 200 ft; the remaining 1,800 ft is open hole. Fig. 2 shows a dual-lateral completion with ICDs, swell packers, and ICVs. The ICDs are spaced 80 ft apart in the 1,800 ft of open hole; therefore, 25 ICDs are installed in each lateral.
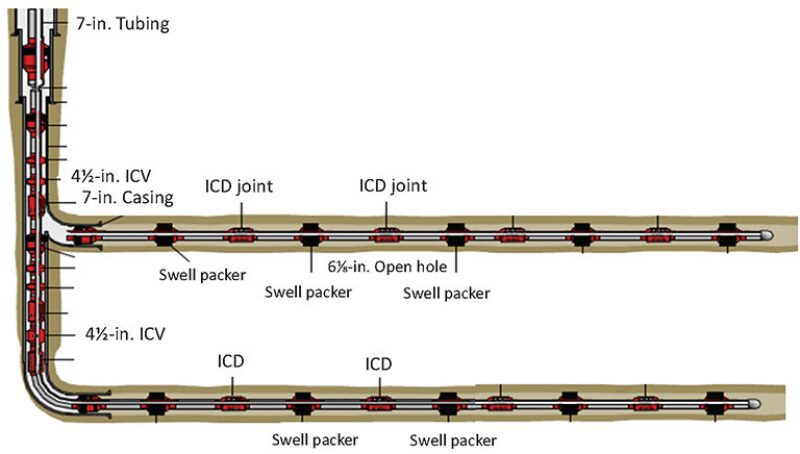
Well-Performance Analysis
For a reservoir with the presented characteristics, a vertical well could produce 5,000 STB/D but with a high pressure drop (3,500 psi) that could accelerate water breakthrough to less than 100 days. A horizontal well could produce the same volume rate and decrease pressure drop to almost 1,500 psi. Nevertheless, a multilateral can produce the expected rate but with a pressure drop of close to 800 psi, thereby delaying the water breakthrough to 500 days. Two cases are simulated—the openhole base case and the intelligent-well case.
The 1,800-ft horizontal section was segmented into six segments: Segment 1, 13,500–13,730 ft; Segment 2, 13,750–14,050 ft; Segment 3, 14,065–14,545 ft; Segment 4, 14,450–14,840 ft; Segment 5, 14,850–15,130 ft; and Segment 6, 15,150–15,400 ft.
Openhole Multilateral Well (Base Case). The base case has the same multilateral trajectory as shown in Fig. 2 but with an openhole completion (no ICVs, ICDs, or swell packers).
Water-Cut Behavior Over Time. For the openhole completion, the SS simulator is coupled to the numerical simulator to explore how water encroaches over time and across segments. Water reached Segments 2 and 6 at 180 days, triggering a very heterogeneous water profile that is difficult to control. Water breakthrough is reached in the rest of the lateral section at approximately 1,500 days.
Multilateral Well Controlled by 50 ICDs. In this case, each segment is isolated using swell packers.
At the initial production, the ICDs have a clear effect on well performance. In those segments with high permeability values, the oil influx was 9 STB/D/ft and pressure drop was negligible. Using a combination of 25 ICDs and six packers, the production profile is now quite homogeneous; therefore, the productivity in Segments 3 and 5 has increased from 1 to 3 STB/D/ft by increasing the pressure drop from 20 to 100 psi.
Water-Cut Behavior Over Time. Water reached Segments 2 and 6 at 800 days, triggering a better homogeneous water profile compared with that of the base case. Water breakthrough is reached in the rest of the lateral at approximately 1,500 days.
Transient Simulation
Fig. 3 plots oil-production and water-cut behavior at the wellhead for the entire multilateral well over time for both (a) open hole (red solid and dashed lines) and (b) the ICD completion (oil rate=green, water cut=blue). In the open hole, water breakthrough irrupts at 20% water cut, reducing oil production from 5,000 to 4,200 STB/D. In contrast, the ICD completion delayed water cut from reaching the 20% breakthrough until approximately 1,000 days.
For the initialization period, the water saturation is 0.25. At 500 days, high-water-saturation zones are observed around the heel and toe of the lateral section, which corresponds to those high-permeability segments. Water breakthrough already occurred at 180 days, whereas, for the ICD completion, the water-saturation front has started to increase toward the horizontal wellbore. For the openhole completion, at 1,500 days, the water-saturation front is quite irregular and channelized between high- and poor-permeability zones. In contrast, using ICDs, the water-saturation front along the horizontal section is very good, equalized, and homogenized, presenting smooth water invasion to the whole lateral.
Optimizing Multilateral Production With ICV Completions
As shown in Fig. 2, one ICV is placed in each lateral. The ICD case was included in an automated optimization process that changes the valve settings over time to maximize oil production, thereby minimizing water production (ICD and ICV are not coupled). The objective function for the optimizer is maximizing oil production while water is reduced. The ICV settings are manipulated by reducing the valve orifice. A setting of 1 means the valve is totally open to flow, and a setting of 0 means the valve is closed. The optimizer gradually reduces the orifice until the objective function is reached. The main variable for the optimizer is the valve setting over 8 years (it is assumed that the ICV setting is changed monthly).
Because there are more than 103 possible combinations for these valve settings, the optimizer was set up to find the optimal solution for dozens of simulation runs to reduce computing time. When the objective function is achieved and penalties are minimized, a global optimum point is determined as the best unique solution.
Conclusions
Coupling a numerical reservoir simulator with an SS completion simulator for a multilateral well revealed significant advantages in simulating the effect of the completion on the reservoir-fluids performance. The design of intelligent completions for multilateral wells could be accomplished with good accuracy when coupling the simulators.
Additionally,
- The coupled model demonstrated that the intelligent completion can delay water breakthrough for twice as long as the openhole completion.
- The study shows excellent improvement in oil recovery and reduction of water production by use of intelligent completions, and shows considerable extension of the producing lifespan of multilateral wells.
- The coupled model demonstrated that multilateral wells with intelligent completions can add up to 1.02 million STB of oil production by reducing water production to 2.03 million STB, less than the openhole completion.
- The extra oil is achieved by installing 50 ICDs (25 along each lateral section) and an ICV in the heel of each lateral.
- The optimization process demonstrated that using ICVs and changing their settings monthly on both laterals can add up to 0.51 million STB of oil and reduce water production by 20%.
This article, written by Editorial Manager Adam Wilson, contains highlights of paper SPE 164815, “Coupling Reservoir and Well-Completion Simulators for Intelligent Multilateral Wells: Part 1,” by G.A. Carvajal, N. Saldierna, M. Querales, SPE, K. Thornton, SPE, and J. Loiza, Halliburton, prepared for the 2013 SPE Europec/EAGE Conference and Exhibition, London, 10–13 June. The paper has not been peer reviewed.