The ideal carbon dioxide (CO2) thickener would be an affordable, safe, water-insoluble additive that could dissolve in CO2 at typical wellhead and reservoir conditions during CO2 enhanced oil recovery (EOR) and elevate the viscosity of CO2 to the same value as that of the oil. Further, the additive would not require heating or an organic cosolvent to achieve dissolution. In this paper, a strategy for designing a novel small-molecule CO2 thickener is detailed.
Introduction
Despite its longstanding success as an EOR technique, CO2 flooding does not recover all of the oil in the formation regardless of whether the reservoir has been waterflooded previously. Typically, primary recovery results in the production of approximately 5–15% of the original oil in place (OOIP), while secondary recovery is responsible for an additional 20–40% of OOIP.
The fundamental causes of this disappointingly low oil recovery can be traced to the density and viscosity of dense CO2. First, the low density of high-pressure CO2 relative to oil promotes gravity override of the CO2, reducing oil recovery in the lower portions of the formation. Second, the viscosity of dense liquid or supercritical CO2 at typical CO2-flooding conditions is approximately 0.05–0.10 cp, a value so much lower than typical oil- and brine-viscosity values that it results in an unfavorable mobility ratio. This leads to viscous fingering, which in turn leads to early CO2 breakthrough, high CO2-usage ratios, delayed CO2 production, depressed oil-production rates, and low-percent OOIP recovery. These problems can be worse when the injection well is completed in two or more producing zones.
The density of CO2 is essentially a function solely of temperature and pressure, and the mitigation of gravity override must be accomplished by mobility or conformance control. It is possible, however, to alter the mobility of dense CO2 by reducing its relative permeability through water-alternating-gas-injection strategies or by generating CO2-in-brine foams. It is also possible to favorably alter the distribution of the injected CO2 in a layered formation, especially if the injected fluids are diverted from high-permeability, watered-out thief zones into lower-permeability, oil-rich zones. Although conformance control is achieved to some extent by each of the mobility-control strategies, there are also techniques designed specifically to block high-permeability zones effectively.
The objective of this paper is to present the initial results of our renewed investigation of another mode of mobility control: CO2 direct thickeners. The longstanding objective of this research has been the identification of a compound that will readily dissolve in dense CO2 at typical CO2 EOR conditions, forming a thermodynamically stable, transparent, single-phase solution capable of flowing through porous media with a viscosity comparable with (or slightly greater than) that of the oil being displaced. The viscosity of the CO2-rich fluid could be controlled readily by changing the concentration of the thickener in CO2.
There are two fundamental strategies for increasing the viscosity of a solution: (a) the dissolution of polymers or associating polymers or (b) the dissolution of small self-assembling compounds that dissolve in the solvent and form viscosity-enhancing supramolecular structures in solution.
There is a long history of attempts to increase the viscosity of CO2 with either polymers or small molecules, which has been summarized in several recent reviews. A concise summary of these attempts, along with discussions of several new reported attempts, is provided in the complete paper.
Designing a Small-Molecule CO
2
Thickener
Our design principles make use of a relatively inexpensive CO2-philic segment (an oligomer or low-molecular-weight polymer that readily dissolves in liquid or supercritical CO2 at conditions commensurate with CO2 EOR), functionalized with CO2-phobic self-associating moieties that promote the formation of viscosity-enhancing supramolecular structures in solution.
Examples of CO2-Philic Materials. Fluorination of the solute typically aids in its CO2-philicity, but it adds cost and environmental liability. To mitigate this problem, we decided to use alternative CO2-philic materials. The existence of specific carbonyl oxygen/CO2 interactions while minimizing solute self-interaction makes these oxygen-containing groups particularly advantageous in CO2-phile design. In addition to weak solute self-interactions and oxygen/CO2 interactions, high flexibility/low-softening-temperature compounds have the potential to enhance entropy of mixing of the solute in CO2. Free volume can also be elevated through branching, methylation, and the use of ether linkages in the backbone. It has been noted previously that most of the CO2-philes known exhibit relatively weak self-interaction, as evidenced by low cohesive-energy density. Finally, short n-alkyl chains are soluble in CO2 and serve as inexpensive CO2-philes because of their low self-interaction strength. Therefore, they can dissolve in CO2, while at higher molecular weights the poor entropy of mixing dominates and the long-chain alkanes do not dissolve in CO2. Thus, our leading candidates for the CO2-philic portion of the molecule include oligomers or low-molecular-weight polymers of propylene oxides or dimethyl siloxane, and short alkyl chains.
Examples of CO2-Phobic Associating Groups. When considering the design of the relatively CO2-phobic portion of the thickener molecule responsible for viscosity-enhancing intermolecular associations, the key to increasing the viscosity of CO2 under any conditions is to create the reality or the thermodynamic illusion of a high-molecular-weight solute at sufficient concentration in a one-phase system with use of CO2 alone as solvent. Given that we have previously ruled out the use of high- and ultrahigh-molecular-weight polymers, we have decided to explore ways in which to create the thermodynamic illusion of high molecular weight in solution through the creation of associative networks built with small molecules, using noncovalent interactions such as hydrogen bonding. While the creation of noncovalent networks in CO2 has been explored previously, it is our hypothesis that those previous approaches have failed primarily because the candidate molecules used associating groups with such strong solute/solute interactions between themselves that CO2 could not dissolve efficacious amounts under traditional CO2-flooding reservoir conditions. In general, we intend to explore the use of aromatic groups because they are not highly CO2-phobic and have been shown previously to induce viscosity-changing intermolecular associations.
For a discussion of thickener candidates, please see the complete paper.
Experimental Methods
Solubility Measurement. The solubility of the thickeners in CO2 was determined by a standard, nonsampling technique. A mixture of CO2 and thickener of specified overall composition (1 wt% concentration of thickener in CO2) was introduced to a high-pressure, windowed, variable-volume view cell retained within a constant-temperature air bath. The mixture was retained in the sample volume above a floating piston in the cell and then compressed and stirred (2,500 rev/min, slotted-fin impeller) at an elevated pressure of approximately 10,000 psia (approximately 69 MPa) at the temperature of interest until a transparent, single-phase solution was attained. The entire sample volume was visible to the observer during this process, and opposing windows allowed the observer to see directly through the sample volume to the light source behind the cell. The mixture was then expanded very slowly by the withdrawal of the overburden fluid situated below the floating piston until a second phase appeared. Typically, a solute-rich cloud point was observed in the form of a fine mist of droplets throughout the sample volume. In order to provide a reproducible and conservative measure of solubility, the cloud point was reported as the pressure at which the suspended droplets prevent one from seeing through the solution, as opposed to the lower pressure at which a greater proportion of the solute comes out of solution and the sample volume becomes completely opaque. After repeating this measurement five times, the temperature of the air bath was raised until the pressure of the solution reached an equilibrium point. The whole procedure was then repeated at the new temperature of interest.
Falling-Cylinder Viscometry. Viscosity data were generated by use of simple falling-cylinder viscometry. An aluminum cylinder was placed within the sample volume of a hollow quartz tube (Fig. 1). Rapid inversion of the high-pressure cell initiated the fall of the cylinder through the single-phase solutions. The cylinder’s outer diameter is slightly less than the inner diameter of the quartz tube used, which reduces the terminal velocity of the falling cylinder and minimizes the acceleration length required to reach terminal velocity. Dimensions of the quartz tube under pressure are kept constant because of pressure equilibration across the tube walls.
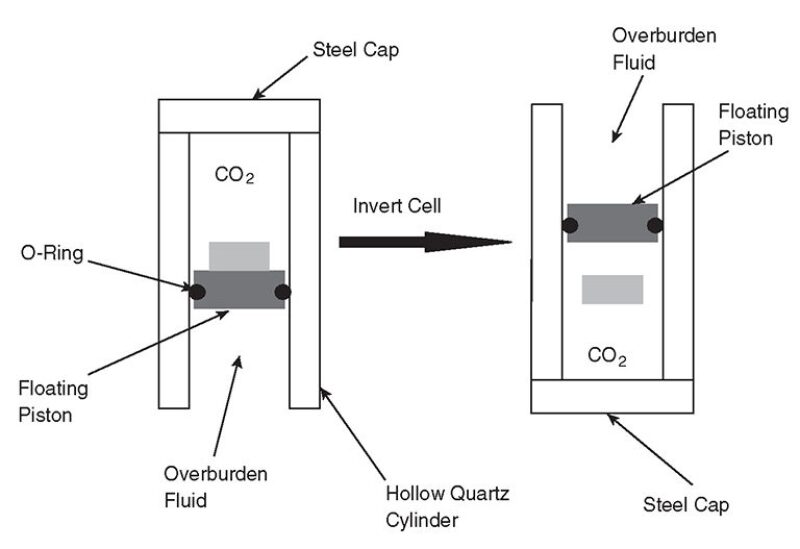
The aluminum cylinder was placed in the cell before the addition of CO2 and thickener. The pressure was then elevated above the cloud point, resulting in a single-phase solution. The cell was then inverted and the time required for the cylinder to drop a distance of 5 cm was recorded. Each measurement was repeated 10 times. The cylinder reached its terminal velocity within several millimeters of travel.
The resulting ratio of the terminal velocities of the cylinder in solution to those in neat CO2 is approximately equal to the ratio of the viscosities under the assumption that the dilute solution is Newtonian and the change in CO2 density induced by the thickener is small.
Solubility Results
Solubility screening for all compounds was conducted at temperatures of 25, 40, and 60°C. The viscosities of the solutions were evaluated at 1 wt% and 25°C.
In general, all of the aromatic-functionalized molecules were soluble in CO2 with the exception of A1 (for a table detailing these molecules, please see the complete paper). This insolubility of A1 can be attributed to the high concentration of CO2-phobic aromatic in the molecule. More than half of A1’s chain has pendant propylbenzene groups; these are CO2-phobic and diminish solubility.
A5, an outstanding water thickener, required relatively high pressures in order to dissolve because the polyethylene glycol (PEG) tails used to enhance water solubility are only slightly soluble in CO2. The modified versions, A5 and A6, use higher contents of polypropylene glycol (PPG), which is more CO2-philic than PEG. Not surprisingly, the cloud-point pressure (a measure of solubility in CO2) decreases as the PPG content increases. A8 required an elevated softening temperature before any dissolution could be achieved.
The carboxylic-acid-terminated polydimethyl siloxane (PDMS) chains, B1–B6, exhibited very low solubility, if any, in the range of pressures tested. The only acid-functional candidate that showed any promise was B1, which had a much longer CO2-philic chain compared with the rest of the B group. B1 still required elevated temperatures to achieve solvation, presumably because of hydrogen bonding’s temperature-dependent behavior.
Viscosity-Enhancement Results
If the compound was soluble in CO2, the viscosity of the solution was assessed at a concentration of 1 wt% at 25°C. The pressure was maintained at a value of approximately 5,000 psi or 1,000 psi above the cloud-point pressure, whichever was greater, and the mixture was stirred at 2,500 rev/min for 15 minutes in order to ensure that a single-phase solution was attained. None of the tested compounds could increase the viscosity under these conditions.
Future Molecular-Design Principles
The structures of these compounds (and others not shown) are being modified continually in an attempt to realize a CO2 thickener. For example, one can use either low-molecular-weight PPG or PDMS as a CO2-phile. In either case, the molecular weight can be increased to make the thickener more CO2-philic if the nature and number of CO2-phobic groups remain constant. However, if the molecular weight of the PDMS or PPG is increased too much, the thickener will begin to lose CO2 solubility because of entropic effects of the polymer alone. Therefore, there is expected to be an optimal molecular weight of the CO2-phile for each thickener.
This article, written by JPT Technology Editor Chris Carpenter, contains highlights of paper SPE 169039, “Development of Small-Molecule CO2 Thickeners for EOR and Fracturing,” by J.J. Lee, S. Cummings, A. Dhuwe, R.M. Enick, and E.J. Beckman, University of Pittsburgh, and R. Perry, M. Doherty, and M. O’Brien, General Electric Global Research, prepared for the 2014 SPE Improved Oil Recovery Symposium, Tulsa, 12–16 April. The paper has not been peer reviewed.