The urgent need to address climate change has unleashed a wave of innovative ideas on how to mitigate the greenhouse gas (GHG) emissions that are driving the problem. Among the approaches considered to hold great promise is carbon dioxide removal, or what some call negative emissions technologies.
These strategies have attracted significant attention in recent years and involve removing carbon dioxide (CO2) from the atmosphere and storing it in long-term sinks such as oceans, forests, and underground geological formations.
What follows is a closer look at how these strategies work, their potential to address the global climate crisis, and the challenges they currently face.
Direct Air Capture (DAC)
This technology captures CO2 directly from the air using chemical reactions and/or physical processes. The captured CO2 can then be stored in a long-term sink, used for food processing and cement manufacturing, or combined with hydrogen to create synthetic fuel. There are currently about 20 direct air capture plants operating worldwide.
Climeworks’ Orca plant in Iceland, the largest operating DAC plant in the world, has the capacity to remove 4,000 tons of CO2 per year and store it deep underground. The majority of commercial direct air carbon capture techniques utilize large fans to force ambient air through a filter, with a liquid solvent, often amine-based or caustic, being used to absorb CO2 from the gas.
There are three chemical processes that are being studied extensively for purposes of DAC: causticization using alkali and alkali-earth hydroxides, carbonation, and organic-inorganic hybrid sorbents consisting of amines supported in porous adsorbents. Additionally, others are exploring membrane separation methods based on semipermeable membranes to capture CO2, which require less water and a smaller footprint.
Perhaps the biggest challenge facing this technology’s ability to scale up lies in its ability to be economically successful given current carbon credit pricing regimes balanced with the unknown amount of energy DAC units will need to achieve meaningful capture volumes.
Ocean Fertilization
The aim of ocean fertilization (OF) strategies is to enhance certain biological processes in the oceans that will lead to increased uptake of atmospheric CO2 followed by permanent storage in deep oceanic layers.
The technique involves the addition of nutrients such as iron, phosphorus, and nitrogen to the ocean for stimulating the growth of phytoplankton that absorb CO2 from the atmosphere. When the marine species die, they sink to the ocean floor, taking the absorbed CO2 with them, where it is stored permanently.
However, the implementation of OF has not been thoroughly studied and there are limited long-term experiments. Additionally, certain OF storage approaches are subject to reversibility. In other words, the stored carbon may not remain sequestered permanently and could be released back into the atmosphere due to the constant changes in ocean conditions such as temperature or nutrient availability.
This issue highlights the need for caution and thorough study before implementing OF as a carbon dioxide removal strategy. The Convention on Biological Diversity and the London Protocolhave called for appropriate regulations and assessments of risks and impacts related to climate‑related geoengineering and OF.
Enhanced Weathering
This approach involves land- or ocean-based methods that accelerate natural geological weathering to remove CO2 from the atmosphere and permanently storing it in solid minerals or ocean alkalinity.
In-situ carbonation of silicates by spreading finely ground silicate rock, such as basalt, over large areas of land is an example of a land-based enhanced weathering technique. Ocean-based techniques involve increasing alkalinity by grinding, dispersing, and dissolving minerals like olivine, limestone, and silicates to address ocean acidification and sequester CO2.
Another approach is in-situ mineral carbonation that involves injecting CO2 into silicate rock formations to promote carbonate formation underground.
While enhanced weathering has the potential to be effective in capturing CO2, it also has the potential to result in negative side effects including unexpected shifts in water and soil chemistry, the release of heavy metals, alterations in carbon and nutrient cycling, and high energy and resource use associated with mining and transporting rock materials. The impact of such scenarios on aquatic and terrestrial ecosystems may need to become a key consideration for project developers.
Biochar
This approach to carbon removal comes in the form of a stable charcoal produced by heating organic material (such as agricultural waste or forestry residues) in the absence of oxygen. Chief processes employed here include slow pyrolysis, fast pyrolysis, gasification, or torrefaction.
When biochar is added to soil as a soil amendment, it can help to sequester carbon for long periods of time, decades to thousands of years, which has made it an attractive option for CO2 removal and storage.
Biochar also has the potential to improve crop productivity through improved soil fertility and water retention capacity. However, one notable concern is the energy and emissions associated with the production of biochar, particularly if fossil fuels are used to power the pyrolysis process.
Soil Sequestration
This strategy refers to the storage of atmospheric CO2 in soil organic matter. Certain farming practices, such as no-till farming, cover cropping, and crop rotation, can increase the amount of carbon stored in soil.
Generally, practices that promote plant growth, reduce soil disturbance, and increase soil organic matter are effective in capturing CO2 in soil. Increasing soil carbon can enhance soil fertility, improve water retention, reduce erosion, and increase biodiversity.
Environmental Impacts of CO2 Removal
Carbon dioxide removal technologies have the potential to play a vital role in mitigating climate change. However, as with any large-scale technology, they also have environmental impacts that need to be considered.
One way to assess these impacts is through a life-cycle assessment (LCA), which considers the environmental impact categories such as global warming potential, acidification potential, eutrophication potential, human toxicity potential, ecotoxicity potential, depletion of natural resources, and land use.
Global warming potential measures greenhouse gas emissions, while acidification potential and eutrophication potential assess contributions to acid rain and nutrient pollution in water bodies, respectively.
Human toxicity potential and ecotoxicity potential measure the potential for harm to human health and the environment through toxic emissions, while depletion of natural resources measures the potential for contributing to the depletion of nonrenewable resources.
Finally, land use assesses the potential for converting natural habitats into agricultural or urban areas. By considering these impact categories, we can gain a more comprehensive understanding of the environmental impacts of a product or process over its entire life cycle.
As we work to address the urgent challenge of climate change, it is important to expand our understanding of LCAs beyond GHGs alone. While GHG emissions contribute to climate change, non-GHG emissions can also have negative impacts on the environment and human health, causing air pollution, acid rain, smog, and more.
That’s why it’s important to assess removal technologies on a wide range of non-GHG environmental indicators, such as soil quality, biodiversity, water consumption, and metal scarcity. By doing so, we can ensure that we’re not shifting environmental burdens from one area to another but are truly creating a sustainable future.
True assessment of environmental impacts requires a cradle-to-grave approach instead of cradle-to-gate approach. In the context of carbon dioxide removal technology, cradle-to-grave assessment refers to a comprehensive approach that considers the entire life cycle of a carbon dioxide removal technology, from its development and implementation (cradle) to its end-of-life management or disposal (grave).
This approach includes all the stages of a technology’s life cycle, such as design, construction, operation, and decommissioning, and considers the environmental impacts associated with each stage. The cradle-to-grave perspective provides a more complete picture of a technology’s environmental impact than a cradle-to-gate approach, which only considers the environmental impacts associated with the production and implementation of the technology and not its end-of-life impacts.
Accounting for the Side Effects
While the most reliable technologies with low uncertainty and limited side effects are soil carbon sequestration (SCS) and biochar, OF is likely to have the largest non-GHG burden due to its elevated level of uncertainty and unknown side effects. Additionally, the multifunctionality of a product system should also be accounted for, as biochar and SCS systems often provide multiple product functions.
While most carbon dioxide removal technologies cause various side effects, DAC appears to cause fewer harmful environmental side effects compared to other technologies. Therefore, the development and implementation of DAC systems could be a viable option for achieving net-zero emissions while minimizing harmful environmental side effects.
Afforestation and reforestation have the potential to be sustainable and scalable options for carbon dioxide removal. Although, the effectiveness of these methods is dependent on available resources like land, water, and nutrients.
Overuse of these resources can have negative impacts on sustainable development and result in negative effects on food production, biodiversity, social cohesion, and human rights.
In conclusion, carbon dioxide removal technologies offer a range of options for reducing atmospheric carbon levels, but their effectiveness and sustainability depend on several factors, such as their durability, scalability, and potential side effects.
While some of these have the potential to be sustainable and scalable, e.g., afforestation and reforestation, they also face constraints related to land, water, and nutrient resources. On the other hand, methods such as DAC have lower risks of leakage and higher durability. However, their energy consumption and potential physical effects on the environment require further research and evaluation.
Ultimately, a comprehensive approach that combines carbon removal technology with other climate change mitigation strategies is necessary to address the overall challenge.
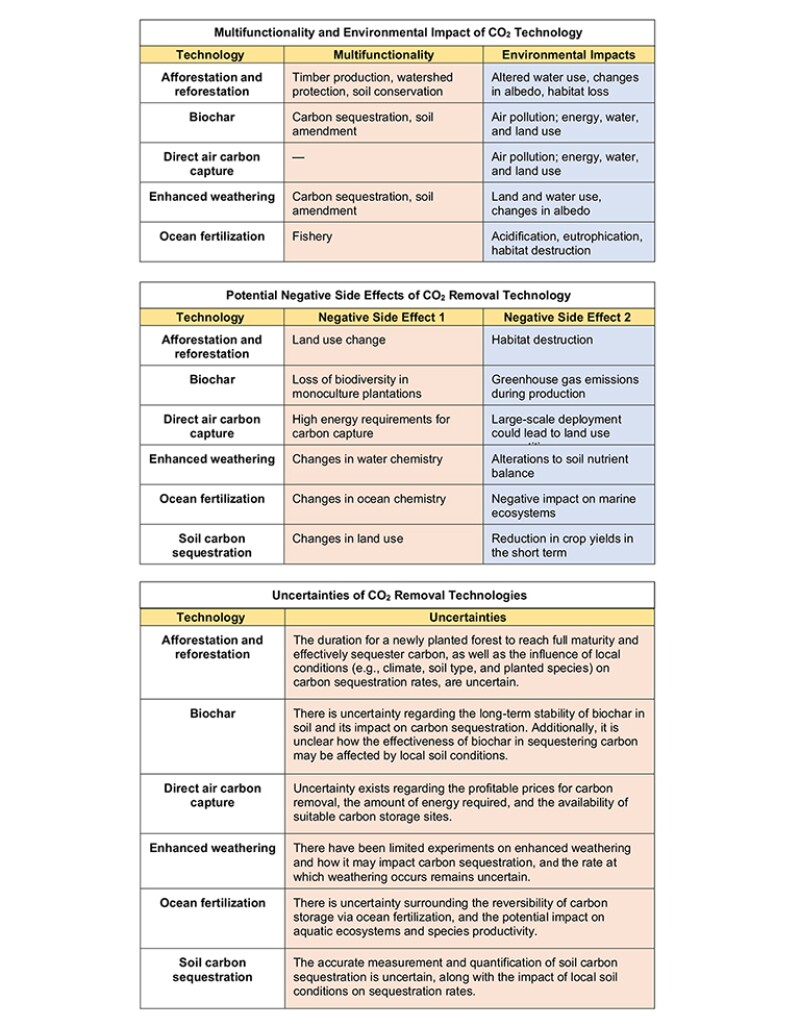
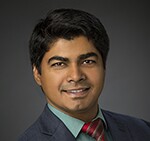
Siddharth Misra, SPE, is an associate professor at Texas A&M University who has published two books and developed nine technologies related to machine learning and electromagnetic sensing for energy and earth resource exploration. In 2018, he received the US Department of Energy Early Career Award, and in 2020 he was honored with four international awards for his contributions to exploration geophysics and subsurface engineering. Misra holds a bachelor’s of technology degree in electrical engineering from the Indian Institute of Technology, Bombay, and a PhD degree in petroleum and geosystems engineering from The University of Texas at Austin. He can be reached at misra@tamu.edu.
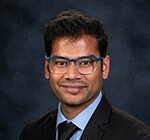
Sateesh Kumar Puri is a civil engineer with a master’s of technology degree in structural dynamics in earthquake engineering from the Indian Institute of Technology Roorkee and is currently pursuing a master’s of science in construction engineering and management from Texas A&M University. His track record includes successfully leading the development of the Chennai Metro Rail Project Phase I, resulting in significant reductions in rework and project delays. Additionally, his work on academic projects such as seismic design of buildings with irregular configurations, analysis of proposed construction planning of a state highway, and design of refugee camps in Turkey, demonstrates his commitment to the advancement of sustainable engineering practices.