In this paper, the authors consider the effect of water chemistry on water/rock interactions during seawater and smart waterflooding of reservoir sandstone cores containing heavy oil. Oil recovery, surface-reactivity tests, and multicomponent reactive-transport simulation were conducted to understand smart waterflooding better. The authors determine that lowering magnesium-ion (Mg2+) concentration results in lower additional oil recovery, and that lowering calcium-ion (Ca2+) concentration leads to higher additional oil recovery.
Introduction
“Smart water” (SW) can be defined as a water engineered by manipulating the ionic composition (adding or removing ions) regardless of the resulting salinity. An upward pH shift has been reported in the literature during low-salinity (LS) and/or smart waterflooding.
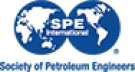